Star Formation Regions (SFRs) --
(see wikipedia definition)
- Useful formulas
-
Basic concepts
-
Turbulence, Kolmogorov
microscales
-
Dynamic viscosity, Kinematic viscosity, Eddy viscosity, Origin of viscosity
-
Sound speed, Thermal line width, Alfven speed, Mach number.
-
Jeans instability, Jeans length, Jeans
mass
, Bonnor-Ebert mass and radius .
- Virial Theorem, Virial parameter, free fall timescale
- Ambipolar diffusion
- Outflow related:
-
Reviews on SFR
-
Observations of SFRs
- Theory and modeling of SFRs
- Chemistry issues in SFRs
- Catalogues of SFRs
- Examples of SFRs
- Some other notes on SFRs
|
Useful fomulas (back to top)
- Dust continuum flux (optically thin) =>M(H2) or Ncol(H2)
or
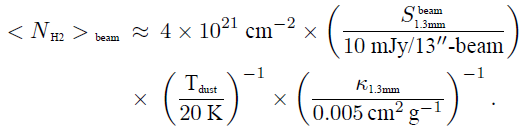
where S^beam_1.3mm is the observed 1.3mm dust continuum flux, Omega_beam is the beam size, mu is the average molecular weight (=2.33), m_H is the H atom mass, kappa_1.3mm is the dust absorption coefficient per unit H2 gas mass = 0.02, 0.01, 0.005 cm^2g^-1 for circumstellar disk around class II YSOs, circumstellar envelope around class I and 0 YSOs, and pre-stellar dense clumps/cores, respectively, B_1.3mm(T_dust) is the Planck function, T_dust usually =10-20 K or even as high as 30K in warm clouds. (see eg. Motte et al., 1998A&A...336..150M)

or for the usual case of 1.3mm continuum observations:
where D is distance, the dust emission is assumed to be optically thin, and Reighley-Jeans approximation has been applied. To scale this formula for other wavelengths, one needs to consider the dependence of the dust opacity on the distribution of grain size, composition and shape. The real SED may not follow a single temperature Planck function but an empirical power law like F_nu ~ nu^-alpha at long wavelength regime where Reighley-Jeans approximation is valid. Here alpha is flux density spectral index that is related to dust opacity spectral index beta (kappa_nu ~ nu^-beta) via: alpha = beta + 2 under the Reighley-Jeans approximation. According to Kwon et al., 2009ApJ...696..841K, beta ~ 1 for class 0 objects (indicating grain growth), thus kappa_nu at other frequencies can be scaled from 1.3mm value as kappa_nu = kappa_1.3mm * (1.3mm/lambda). See a similar formula also in Motte & Andre, 2001A&A...365..440M.
- 12CO, 13CO line flux densities=> Ncol(H2) => M(H2) => AGB mass loss rate
(see, eg., Guan, Wu & Ju, 2008MNRAS.391..869G)
- First, determine the 13CO optical depth:
Here 12CO line is assumed to be completely optically thick while 13CO optically thin. A similar formula can be used for C18O.
- Second, determine the 13CO column density:
where the common excitation temperature Tex = Tmb(CO2-1) * theta_s^2 / (theta_s^2 + theta_b^2), theta_s and theta_b are the source and beam diameters respectively. Or alternatively, Tex can be determined by 13CO 2-1 and 1-0 line ratio. B=57635.968 MHz, mu=0.11011 Debye are rotation constant and magnetic dipole moment of 13CO respectively, J is the quantum number of the lower state of the transition. (Note: here a beam filling factor of 1 is assumed in above formula. But in reality, the 13CO or C18O regions might be smaller than the beam and thus the filling factor can be much smaller than 1!)
- Third, determine the H2 column density: Ncol(H2) = 10^4 * 89 * N(13CO ), or Ncol(H2) = N(13CO) / 1.7x10^-7, where the cosmic 12CO abundance [12CO/H2] = 10^-4 and solar isotopic ratio [12CO/13CO] = 89, and abundance of C18O of 1.7 x 10^-7 (Frerking et al., 1982ApJ...262..590F) are assumed.
- Fourth, determine the gas mass:
where the source radius R can be calculated when the distance and angular size of the 13CO region are known.
- Last, in the case of spherical expanding envelope around an AGB star, mass loss rate can be estimated: Mlr = M(H2) * Vexp / R.
- line width => virial mass
- M_vir = 3*R*sig^2/G = 697.5 Msun * (R/pc) * (sig/km s^-1)2 = 126 Msun * (R/pc) * (FWHM/km s-1)2, where sig = FWHM / sqrt(8 ln2) is the velocity dispersion of a cloud. Sig includes three parts: thermal broadening (= kT/mu/mH), turbulent broadening, and variation of clouds at different l.o.s. in the beam. (Literature?)
- (from Wikipedia: Virial_theorem): Approximately, when the gravitational potential and internal energy equals, we have 3/5 GM/R = 3/2 kT/μmH=σ3D2/2=3/2 σl.o.s.2, where R is the radius of a spherical cloud, σ3Dand σl.o.s. are 3D and line-of-sight velocity dispersions of the considered molecule, respectively . Thus, the Virial mass is
Mvir=5/2 R σl.o.s.2/G.
- The formula from Bertoldi & Mckee (1992ApJ...395..140B): Mvir = 5 R σl.o.s.2/G. This is a factor of 2 larger than in that inferred from the wikipedia. But the difference is not important because the equation is only roughly correct.
- V_LSR => Distance
- Using the mordern parameters of Galactic rotation: R0 = 8.4+-0.6 km/s, Theta0 = 254 +-16 km/s and new peculiar motion of the sun, kinematical distance can be calculated from measured V_LSR using the Fortran code provided by Reid et al., 2009ApJ...700..137R: apj309648_revised_kd.tar One only needs to fill in source data in source_file.dat and round the Fortran, the D_k and corrected V_LSR can be find in the output file example_print.out.
Basic concepts
(back to top)
-
Turbulence -- (see its wiki entry here) turbulent
flows that can be roughly statistically described by Kolmogorov
microscales. Velocity increment du(r) = u(x+r)-u(x) is useful. According
to statistical scale-invariance and the Kolmogorov 1941 theory, we have the structure function in turbulence as
<du(r)^n> = Cn * epsilon^(n/3) * r^(n/3),
where <> denotes statistical average and Cn would be universal
constants. However, the structure function has been found to deviated from
experimental data when n is large. Thus the Kolmogorov 1941 theory is not
exactly correct and is now under revision.
-
Kolmogorov microscales -- (see its wiki entry
here) With
the simple idea that the smallest scales of turbulence
are universal and that they only denpend on the average energy dissipation
rate epsilon and the kinematical viscosity nu of
the fluid, A.N. Kolmogorov obtained his
microscales by unit analysis under mean field assumption: (the unit of nu is length^2/time, that of
epsilon is length^2/time^3.)
- Kolmogorov length scale
-
Kolmogorov time scale
-
Kolmogorov velocity scale
With this idea, only the large-scale eddies are dependent on flow
geometry, while smaller-scale eddies are self similar
at different size scales, which stimulated the large eddy simulation
algorithm in which the smaller-scale eddies can be treated as eddy viscosity.
-
Dynamic viscosity -- mu = tau/(du/dy), the ratio of stress to veocity
gradient, where y is the axis perpendicular to the velocity. The unit is
[Pa.s] = [kg/m/s]. Relation to free path of diffusing particles lambda: mu =
2 rho * ubar * lambda, where rho is fluid density, ubar is average molecular
speed.
-
Kinematic viscosity -- nu =
mu/rho, the ratio of dynamic viscosity to
fluid density. The unit of nu is [m^2/s]. Relation to free path of diffusing
particles lambda: nu = 2 ubar * lambda.
-
Eddy viscosity -- a quantity used to
characterize the transport and dissipation of energy in the smaller-scale
flows in a large eddy simulation. With the eddy viscosity, one does not need
to deal with smaller-scale eddies.
-
Origin of viscosity: for gases, the viscosity originates from molecular diffusion, while for liquids,
the main contribution of viscosity comes from molecular
forces, although the molecular diffusion is still there as a minor
contributor.
-
The speed of sound -- see the wikipedia
definition here.
-
In a general sense, c=sqrt(C/rho), where C is a coefficient of stiffness and rho is the density.
-
For gas, c=sqrt(gamma * p / rho), where gamma is the adiabatic index or heat capacity
ratio and p is the gas pressure. Gamma ~5/3
(=~1.6667) for monoatomic molecules such as noble gases, ~7/5 (=~1.4)
for diatomic molecules in vibrational ground state, and usually
experimentally measured to be within 1.3991 to 1.403. For more gamma
values for specific pure gases, see wikipedia page here.
-
For ideal gas, c=sqrt(gamma * R * T /
M)=sqrt(gamma * k * T / m), where R~8.3145
J/mol/K is the molar gas constant, T is
absolute temperture in K, M is the molar
mass in kg/mol (~0.0289645 for dry air), k is boltzmann constant, m is the mass of a
single molecule in kg.
-
It also can be written as c^2 = [d_p/d_rho]_ad,
where the differential is partial differential in adiabatic case.
- thermal sound speed: Cs = sqrt(kT/μmH) ≈ 0.2(μ/2.0)^-1/2(T/10)^1/2 [km/s], where μ is mean molecular weight. (from Hennenbelle & Chabrier., 2008ApJ...684..395H)
-
Thermal line width -- Thermal motion speed distribution is discribed by the 3-D Maxwell-Boltzmann distribution (multinormal distribution). It has three characteristic velocities: (wikipedia)
Vmp = sqrt(2 * R * T / M) the most probable speed -- the speed at the maximum of the Maxwell-Boltzmann distribution;
Vavg = sqrt(8 * R * T / pi / M) the average speed -- the speed summed over all particles and divided by the particle number;
Vrms = sqrt(3 * R * T / M) the root mean squre speed -- the root of the average of the squared speed among all particles.
where R is the gas constant, T is the temperature and M is molar mass of the gas (R/M = k/m, where k is the Boltzmann constant and m is the molecular mass). These are 3-D speeds , however.
For observed thermal line width, it reflects the line-of-sight projection of the velocity field -- a 1-D normal distribution with a dispersion of σ = sqrt(R * T / M). Assuming the spectral line of a molecule of weight (mobs) is observed, the expected FWHM thermal line width of this molecule at a kinematic temperature of T can be computed as
FWHM = ΔVobs = sqrt (8 ln2) σ = sqrt (8 ln2 kT/m_obs) = 0.214 km/s * sqrt(T/mobs),
and the average relative motion speed of the mixture gas of this molecule with H2 and He can be computed as
,
where k is the Boltzmann constant and <m> =2.33 amu is the mean particle weight of interstellar gas ([He/H2]=0.18, or one He for every five H2). (See, eg., Mardones et al., 1997ApJ...489..719M). This ΔV0 is useful when computing chemical reaction rates of the molecule with H2 or He. (However, I think the use of the reduced mass of H2+He in this formula doesn't make sense. Instead, we should relace <m> with the mass of H (mH) such that the term in the parentheses above is just the reciprocal of the reduced mass of mobs and mH.)
It can be rewritten as
ΔV0 = 0.214 km/s * sqrt (T * ( 1/m_obs + 1/<m> ) ),
if m_obs and <m> is in amu and T in K. For example, the N2H+ line observed by Mardones et al., 1997ApJ...489..719M should has a thermal line width of FWHM = 0.154 km/s, while the average relative speed is ΔV0 = 0.843 km/s between N2H+ and H2 and ΔV0 = 0.564 km/s between N2H+ and H2+He average atom (does the average atom make sense?) when Tk = 15K. This ΔV0 can be used to compute collision rate.
According to the discussion of the use of reduced mass in wikipedia (link), reduced mass is used in kinetics mainly in the computation of the change of kinetic energy when two molecules inelastically collide and lose kinetic energy to promote chemical reaction between them, say, ΔE = 1/2 <m> Vrel2 (totally inelastical).
- Alfven Speed -- VA=B/sqrt(4πρ) for single ion species, where B is the magnetic field strength, ρ is the density of the ionic species. (from Li et al. 2002ApJ...569..792L) (also see the wiki entry)
- Mach number -- Ma = V/Cs, usually used for supersonic motions where Ma > 1. (also see its wiki entry)
- Jeans
instability -- (see its wikipedia entry) When the
internal thermal gas pressure is not strong enough to prevent gravitational collapse
of a region filled with matter, it will occur to cause the collapse of
interstellar clouds and subsequent star formation. The stability requires
hydrostatic equilibrium:
where p is gas pressure, r is the radius of the cloud, G is the gravitational constant, rho is the mass
density, Menc is the mass enclosed in the
cloud. Or in another way, we can consider a perturbation to a cloud in
equilibrium. There are two time scales: sound-crossing time

and free-fall time
where R is the cloud diameter, c_s is the sound speed, G is the gravitational constant, rho is the mass
density of the cloud, n is number density of
the cloud. When tsound < tff, the pressure --
the restoring force -- wins and thus the hydrostatic equilibrium is stable. Otherwise, the gravity wins and the cloud
will begin to contract with free fall time scale untill other supporting
forces become important.
-
Jeans length -- a critical length scale R_J of a cloud when the thermal energy balance the
gravity (in another word, when the sound-crossing time tsound is equal to free fall
time tff). It is also the oscillation wavelength below which stable oscillation
rather than gravitational collapse will occur (see in the Jeans instability on wikipedia).
where k_B is Boltzmann's
constant, T is the temperature of the cloud, G is gravitational constant, r is the radius of the cloud, mu is the mass per
particle (~3.9x10^-24 g for gas composed of four H2 molecules plus one He
atoms), rho is the mass density of the cloud, c_s is the sound speed, n = rho/mu is the number density of the cloud.
-
Jeans mass -- The total mass contained in a spherical cloud of average density ρ and of diameter of Jeans Length (see in the Jeans instability on wikipedia):

where RJ is the Jeans length and cs is sound speed.
- Bonnor-Ebert mass and radius -- They are the maximum mass and radius an isothermal hydrostatic spherical cloud can have to maintain stability against gravitational collapse. The solution was first obtained by Bonnor ( 1956MNRAS.116..351B ) and Ebert ( 1955ZA.....37..217E ) independently. Given a cloud of homogenous temperature T, assume the gas obeys the quation of state of ideal gas
,
where p is the gas pressure, k is the boltzmann constant, ρ is the gas density, T is the gas temperature and m is the mass of a molecule and it's also hydrostatic, the gravity is perfectly balanced pressure gradient as
,
then the radial density profile should be centrally enhanced and the density profile can be written as
,
where λ is an arbitrary constant and ψ is the solution of
,
in which ξ is the normalized cloud radius defined in the formula
,
where
.
The solution to ξ can not be analytically obtained, however. Bonnor ( 1956MNRAS.116..351B) investigated the stability of such a cloud and, with the help of numerical investigation in literature, he obtained the smallest critical radius (at ξ =6.5)beyond which the cloud will lose stability against gravitational collapse -- the Bonnor-Ebert Sphere Radius
,
or in terms of average density as
,
or in terms of density at the critical radius rc as
.
Bonnor ( 1956MNRAS.116..351B) also gave the critical mass corresponding to this critial radius -- the Bonnor-Ebert Sphere Mass
.
At the outer edge (r=rc) of such a spherical cloud, the finite gas pressure P0 can be calculated from the density ρc and cloud temperature T using the ideal gas equation of state. This means the cloud is in equilibium with an ambient pressure of the same strength. The Bonnor-Ebert Sphere Mass thus also can be expressed in terms of ambient pressure P0, as given in wikipedia page (link here)
,
where VT=sqrt(kT/μmH) is the isothermal sound speed (γ=1), and the dimensionless constant CBE≈1.18.
-
Virial theorem --
For a stable system bounded by potential forces, time-averaged total potential energy <Vtot> is 2 time of the time-averaged total kinetic energy <T> , say
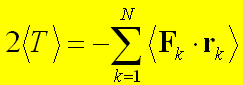
where F_k is the force on the kth particle, which is located at the place r_k.This relation allows the calculation of total kinetic energy for very complex systems and holds for all systems in and out of thermal equilibrium. In the case of thermal gas, the average total kinetic energy can be related to temperature through equipartition theorem. If the force between any two particles results from a potential energy like V(r) ~ r^n, a simpler form of the virial theorem results:
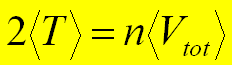
- Virial parameter: It is used to characterise the approximate ratio of kinetic to gravitational potential energy (see in McKee & Zweibel 1992ApJ...399..551M)
,
where sigma_v is the total 3D velocity dispersion (including both thermal and non-thermal motions), R is the radius of the medium, M is the mass and G is the gravitational constant. Alpha = 2 indicates energy equipartition between the two energy terms.
- Free fall timescale. It is only related to gas density (see e.g., Dobbs et al. 2014prpl.conf....3D) :
,
where n_H2 is the number density of H2 molecules and the mass per H2 molecule is 3.9x10^-24 g for a fully molecular mas of cosmic composition.
- Ambipolar diffusion -- In astrophysics, it refers to the decoupling of neutral particles from plasma in the initial stage of star formation (see its wiki). Ions in the clouds (mostly H+ and e-) are tied to interstellar magnetic field. They can hinder the gravitational collapse of neutral particles (mainly H) by collision interaction. However, if the fractional ionization is very low (10^-6 or less), the collapse is not hindered because the collisions are rare.
- Outflow related concepts:
- Collimation factor: Rcoll = D_major/D_minor, where D_major is taken as the maximum size of the outflow, and D_minor = 4 * area / D_major, assuming the outflow as an ellipsoid.(see Bally & Lada, 1983ApJ...265..824B)
- Momentum of the outflow:
, where Mv is the H2 mass at velocity v and a small area, i is the inclination angle to the l.o.s, A is the whole area of the outflow. Angle i is difficult to determine. If assume sin i =1, we get lower limit of the quantities. (from Lada, 1985ARA&A..23..267L)
- Kinetic energy of the outflow:
, where the meaning of i, v, A is the same as above.
- Dynamical time of the outflow: tau_d = R/V, where R is the maximum size and V the velocity of the outflow.(from Lada, 1985ARA&A..23..267L)
- Mass loss rate of the outflow: Mlr = M / tau_d, where M is the total mass of the outflow, and tau_d is the Dynamical time (see above).
- Driving force of the outflow:
.(from Lada, 1985ARA&A..23..267L)
- Mechanical luminosity of the outflow:
(from Lada, 1985ARA&A..23..267L)
|
|
Reviews on massive SFR
(back to top)
- Review by Shu, Adams & Lizano, 1987ARA&A..25...23S: Star formation processes involve 12 orders of magnitude of mass and size scales (10^11 to 10^-1 Msun, 10^23 to 10^11 cm). They reviewed the SF studies on the scale of giant molecular clouds (roughly <10^6 Msun, <10^20 cm).
(back to top)
- IMF: unlike the power law IMF of salpeter (1995), there could be two peaks around 0.3 Msun and 1.2 Msun.
- Induced or triggered star formation requires outside-in collapse of clouds, while spontaneous star formation requires inside-out collapse of clouds. Which is dominant? Observations seem to prefer the latter.
- Fission of clouds occurs in equilibrium, fragmentation occurs in hydrodynamic processes. Binaries with P < 100~1000yr could be formed in a disk system, while binaries with longer P could be formed by fission of clouds or in a cluster.
- Physical conditions:
- Giant molecular clouds (GMCs) usually have masses of 10^5 to 3x10^6 Msun, sizes of several tens of pc.
- GMCs are actually cloud complexes composed of clumps of masses of 10^3 to 10^4 Msun, sizes of 2 to 5pc, densities of 10^2.5 cm^-3, and Tk ~ 10K.
- Clumps have smaller cloud cores of rough mass of ~1 Msun, size of ~0.1 pc, and Tk ~ 10K.
- Large fraction of mass should be contained in dwarf molecular clouds (DMCs), while GMCs has short lifetime and thus could be trantient objects.
- Because the Jeans masses are far smaller than the clump masses, the clumps should be mechanically ballanced, otherwise, free-falling clumps might result in far too high star formation rate in the Galaxy. The major support agents include magnetic fields, rotation and turbulence.
- The critical mass of a molecular cloud below which the cloud can be supported by a magnetic field of magnetic flux Phi is

or equivalently a critical column density
(e.g., Field 1970)
or equivalently a critical visual extinction
, assuming canonical gas-to-dust ratio of 20.
However, the magnetic field can not support the cloud along the field line directions. Measurement of dark cloud rotation (e.g. that of Goldsmith & Arquilla, 1995, Fuller & Myers, 1987) showed that the rotation rates are uniformly low and undetectable (< ~1 km/s/pc), indicating that most clouds are subcritical, i.e., they are under control of magnetic braking and thus not contracting rapidly as a whole.
- Thermal balance: The temperature of ~ 10K in the molecular cloud envelopes is determined by the balance between cosmic ray heating and CO line cooling. In the outer part of the clouds, photoelectrons released from dust grains is an additional sources of heating. However, the hotter gas around young OB stars may not all be heated by thermal coupling between dust and gas, but by some other processes, such as ion-neutral collisions in ambipolar diffusion.
- Two modes of cloud contraction:
- Supercritical contraction -- M_cl > M_cr:
- The cloud evolution is characterized by magnetically diluted collapse (Scott & Black, 1980), and the faster contraction along the field lines may result in fragmentation into substructures that are supercritical by themselves.
- With mean magnetic field of B=30uG, when the surface density (column density) is > 80 Msun/pc2 or the Av>4 mag, the cloud should be contracting rapidly.
- A representative chain of objects: Taurus molecular clouds (subcritical with Av~1, low mass SF); rho Ophiuchi molecular cloud (near supercritical with Av~6, low mass SF with a few B star); W3(OH) (supercritical with Av~1000mag, OB cluster).
- The infall tracer, HCO+, is an ion, thus the contraction may freeze the magnetic field inside the gas.
- Ambipolar diffusion, together with thermal pressure, is always a component counteract with gravitational force in all clouds.
- Subcritical contraction --
M_cl < M_cr:
- Cloud
evolution is controlled by ambipolar diffusion. No dynamical contraction can be triggered.
- Although the magnetic field can slow down the gravitational contraction of the clouds through collisions between ions and neutral particles, the neutral material still pass by the ions and slowly contract. Meanwhile, the strong magnetic field in the cloud tends to decay with time to the background value with a very long timescale. Thus, the cloud contraction has a very long timescale under the control of this magnetic decay process, and the SF efficiency is very low.
- However, the clouds still can quasi-statically fragment into small cores that may start faster contraction to form low mass stars. Because of the slow contraction of the whole cloud, the first generation of stars have ample of time to generate outflows to blow away gases around adjacent star embryos and thus only form loosely associated low mass cluster.
- The ion-neutral collisions in the ambipolar diffusion processes might play a role in the cloud heating. This could be the case particularly in those regions of the cloud far from YSOs.
- The initial condition of a cloud before contraction should be a singular isothermal sphere (without rotation or magnetic field):
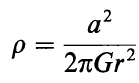
where a = sqrt(KT/m), with T and m being the constant temperature and mean molecular weight of the gas (see, e.g., Chandrasekhar, 1939). Although this density law has an unphysical singularity at the cloud center and an unlimit mass at infinite radius, the observed 1/r^2 density law indicate that it can be an good initial state for the cloud collapse. In this state, with unlimited supply of matter, the cloud has no characteristic mass and thus no characteristic Jeans mass. But it has one Jeans mass at each radius r that determines the stability of the cloud.
- Review by Crutcher, 1999ApJ...520..706C: Magnetic Fields in Molecular Clouds. (to be read...)
- Review by Lada & Lada, 2003ARA&A..41...57L: Eembedded clusters in molecular clouds (to be read...)
- Review by Mac Low & Klessen, 2004RvMP...76..125M: Control of star formation by supersonic turbulence. (to be read ...)
- Review by Zinnecker & Yorke, 2007ARA&A..45..481Z: Massive star formation processes are still not well understood. Large distances, high
extinction, and short time scales of
critical evolutionary phases make observations of the process very
challenging. Theoretical work also remain controversial. They reviewed
three competing concepts of massive star formation: monolithic collapse in isolated cores; competitive accretion in precluster environment (stars move around so as to accret gas at different places, the rich get richer,
the accretion domain of stars at
the cluster center extend to the whole cloud); stellar collisions
and mergers in very dense systems. They also review observed
outflow, multiplicity, and clustering properties of massive stars, the
upper initial mass function and the upper mass limit. They conclude that the formation of massive star is NOT a scale-up of
low mass star formation.
Below are some fast facts from the paper:
(back to top)
- Major questions to answer upon massive SF:
- What is the sequence of observable
states leading from molecular clouds to young high-mass
stars?
-
What are the initial conditions of massive star formation (gas densities, temperatures,clump
masses, etc.) and how do they come about?
-
Do massive stars always form in dense stellar clusters or can they form in isolation? What special conditions are
necessary to allow coalescence,
i.e., mergers of stars?
-
Which clues to the origin can be gleaned from multiplicity observations? How do we
explain the very tight massive spectroscopic binaries and OB
runaway stars?
-
How does the forming massive star influence its immediate surroundings, possibly
limiting its final mass and/or the final mass of its
neighbors?
-
How do young massive stars influence their global environment, either by inhibiting
or by triggering further star formation? How do we get a
starburst?
-
Different stages of the embedded phase
proposed from observations:
- IR dark cloud (IRDC) -- density
maxima with temperature minima. see the compilation of dozens of
IRDCs by Sridharan et al., 2005ApJ...631L..73S.
-
Hot molecular core (HC) -- large
mass of warm and dense gas, large abundances of complex organic
molecules evaporated from dust grains, CH3OH maser.
-
Hypercompact and Ultracompact H II region (HCH II &
UCH II) -- environment is ionized locally. HCH II is probably
related to stars with disk while UCH II may be linked to
disk-less stars.
-
Compact and classic
H II region -- gas is ionized globally, H II region
expands hydrodynamically, clear away the natal clouds.
-
Star formation steps:
- Compression,
-
Collapse -- Jeans mass over which
gravity begins to dominate pressure:
.
Once gravity dominates pressure and
magnetical force, the optically thin lines can effectively
radiates away compressional heat, and thus the collpase proceeds
as free fall with a timescale of
.
The free fall is stopped when the central
core becomes optically thick so that gas pressure rises up fast due
to adiabatic heat up.
-
Accretion -- After the H2 molecules begins to dissociate, a second inside-out collapse will occur (because
all molecules have been dissociated, thus radiation escapes and
gas pressure drops down). Then a quasi-hydrostatic core establishes in the center and
material continues to accumulate on it as long as the inside-out collapse
lasts. Simultaneously, the core contracts with Kelvin-Helmholtz timescale
towards H burning densities and tempertures. A massive star
reach main sequency well before it finishes accretion, and thus
it will evolved along the main sequency
upward in the H-R diagram, untill it has no mater to
accret. This is different from non-accreting massive star
evolution model.
-
Disruption -- The accretion disk
surface will be ionized by UV flux from the star and a disk wind results and interacts with stellar wind to collimate the latter. Therefore, collimated outflow can form even without
the help of magnetic field. The developing H II region might not
stop the accretion, as usually expected, but help the accretion by magnetic braking of
the ionized infalling material (ionized accretion
flow)! Only
when the H II region grows beyond a critical
radius within which the gas is gravitationally bound, the
H II region will burst freely and start to disrupt the dense
clouds.
-
Inital conditions of massive SF: Ncol(H2) = 10^23~10^24 cm^-2, n_H2 ~ 10^5 cm^-3, Tgas ~ 10-20K, diameter ~ 0.5 pc, magnetic field can slow down the set-up for
collapse when the cloud is disturbed by convergent turbulence.
-
End products of massive SF:
- OB clusters -- classified into
three types: clusters that contain ~ 1, ~ 10 and ~ 100 O stars.
However, a super cluster with 4000~6000 O7V stars were found in
the dwarf galaxy NGC 5253.
-
OB associations -- scattered
distribution of OB stars
-
Field OB stars -- 43 of the 227 O
stars brighter than V=8mag are field stars, of which 10-20 stars
can not be tracked back to any known clusters or associations. HD
93521 must be a O9.5V star formed locally at a high latitude!
-
IMF:
- dN/dlog(M) ~ M^x, with x=-1.35
from Salpeter, 1955ApJ...121..161S,
could be still correct at the high mass end up to now.
-
The mass distribution of the pieces in
any hierarchy has a logarithmic slope of
-1. If the sampling rate (the possibility to pick a mass to form a star) is assumed to be propotional to density so as to mimic the
dynamic processes involved in star formation, we can derive a
IMF slope steeper than that of cloud pieces (-1), say, the salpeter slope of -1.35. (For a
fractal cloud, the mass of a cloud piece scales with size to a
power equal to the fractal dimension, while the density of a
cloud piece scales with size to a power equal to the fractal
dimension minus 3. Therefore, smaller pieces have relatively
higher sampling rates due to lower luminosity.)
-
Numerical hydrodynamical calculation of the stellar mass in star burst regions (warm and dusty gas) by Klessen et al., 2007MNRAS.374L..29K predicted a top-heavy IMF, possibly
stemmed from the elevated thermal Jeans
mass due to the higher temperature.
-
They estimated that currently we should have about 1000 accreting massive stars (> 30
Msun) in the Milky Way galaxy.
-
There could be an upper limit of stellar
mass around 150 Msun, but it's not very sure yet. The
old vibrational instability limit of ~ 100 Msun is no longer
correct because it does not have a global disrupting effect. The
upper mass limit from the dust opacity during the accretion
process is incorrect as well, because it's known now that
accretion continue via disk. The ionized gas opacity in the
inner most part of the accretion disk might matter in
determining the upper mass limit, but the opacity is difficult
to assess. Mergers could be another to extend the upper mass
limit, but it also suffer a limit due to depletion of number
density of stars in the cluster center.
-
Post-ZAMS evolution of massive stars:
-
It's possible that a massive star has begun to depart ZAMS when it's still accreting material through disk and losing material through stellar wind. This is dictated by its mass loss rate and
mixing that is induced by rotation.
-
For low luminosity O stars (e.g., dwarf
stars of later O type), the stellar wind and UV flux may
not be strong enough to blow away its natal clouds, and thus
remain embedded for a longer time even after their ZAMS.
-
Binaries and multiple systems:
-
Examples:
-
Theta^2 Ori A (in Orion' bar)
is a hierarchical triple system (massive close binary plus a
distant third star);
-
sigma Ori is a more complex
hierarchical system;
-
theta^1 Ori C is a binary
with highly eccentric orbit;
-
WR22 or HD 92740 is the most massive WR star (Mzams~80Msun)
in the Galaxy, residing in a binary system with P = 80 day.
-
Pismis 24-1, a O2V type
massive star, later being identified to be a visual triple
system.
-
LBV 1806-20 with mass over
200 Msun, possibly being a spectroscopic binary system.
-
Pistol star, the most massive
star (200-250 Msun) in out Galaxy is in Quintuplet Cluster
near the Galactic Center.
-
WR20a, the most massive
double lined spectroscopic binary: 83 and 82 Msun. P = 3.7
day. In the Westerlund 2 cluster associated with RCW49 H II
region.
-
Massive multiple systems in the 30
Dor cluster in the LMC:
R136-38, R136-42, Melnick 34, etc.
- Cold rotating Keplerian disks
produce circular orbit, sub-Keplerian disk rotation or cold filaments tumbling end over end produce highly eccentric orbits.
-
Accretion of gas will shrink the orbit
size of a binary system and thus from compact O star
binaries. Angular momentum of a binary is A ~ M*V x R ~ M^3/2 *
R1/2 (because the Keplerian orbit speed V ~ (M/R)^1/2). If the
accreted gas has zero angular momentum, then A is constant
during the accretion and thus R ~ M^-3; if the accreted gas has
constant specific angular momentum, say, A ~ M, then R ~ 1/M.
The SPH simulation gives R ~ M^-2,
which indicating that the specific angular momentum of accreted
gas is decreasing with time in the SPH model.
-
Mass ratio of binary tends to become
unity if they are formed via accretion, because (1) lower
mass component move faster and thus can accret gas that is
spiraling in along the same direction; (2) swelling of
protostars due to deuterium shell burning causes mass transfer
to the secondary in a close binary.
-
Wide and non-equal mass binaries can
form via disk-assisted capture or fragmentation of disk or filament.
-
In N-body simulations, formation
of a massive binary will use its kinetic energy to kick out low mass stars so as to
successfully shrink its orbit size. This explains many aspects
of observed massive binaries. However, an unexpected
anti-correlation between massive binary and number density of
clusters is observed, which remains unexplained by the N-body
simulation, unless most of the massive binaries have merged into
a single star.
-
Formation of 4 massive stars in Orion
Trapezium system: massive stars form in the center of
subclusters via competitive accretion, then subclusters merge to
form a single cluster containing multiple massive stars.
-
Among the 227 bright O stars (V<8mag), 50 are known spectroscopic binary systems (P<30 days, mass ratio = 0.5~1.0), 42
are wide visual binaries (P = 100~1000days,
mass ratio= 0.5-1.0), leaving a gap of
period in between. This could be a selection effect and
nowaday interferometer could detect them. The binary frequency
is 60% for this sample. (see details in Mason et al., 1998AJ....115..821M)
-
10-25% of O stars are runaway stars, while that for B-type stars is only 2%. The binary
frequency is much lower in O runaways than in clusters.
-
Disks and outflows:
- Some massive stars show less collimated
outflows or no outflows.
Collimated outflows and jets occur only in sources with L <
10^4 Lsun (at most B stars), never in O stars.
-
It's still not clear to what extent the
observed outflow trace the combination of stellar wind and disk
wind (the latter collimates the former and is capable of
entraining surrounding gas).
-
The emission from accretion onto
disk and onto the central star contribute significantly to the luminosity of massive SFRs, thus stellar
mass can not be retrieved from luminosity.
-
Theoretically, the accretion rate is expected to increase when
the central core mass accumulates. However, the outflows are
usually observed to be strongest in the earliest stage of SF,
which indicates that the accretion rate
should decrease with time. It's still unclear how to
reconcile the observations with theories on this point.
-
Masers:
- OH and CH3OH masers are exclusively associated with massive SFRs, because
they need nearby strong IR photon sources to pump them.
-
H2O masers can appear in
both massive and low-mass SFRs, because they are collisionally
pumped.
-
Massive SF is not a scale-up of low and
intermediate mass SF:
- First: Strong UV radiation from massive young star
ionize/dissociate disk and envelope, produce disk wind and
bipolar outflow. The bipolar jets in low mass SFR is boosted by
magneto-centrifugal forces.
-
Second: Massive stars grow along the main sequence by gaining
matter at an acccretion rate of 10^-4 Msun/yr, while low and
intermediate stars has a long pre-MS stage.
-
Third: Massive star need competitive accretion process to gain
enough mass in a cluster.
-
Finally: Massive stars are more capable of triggering
subsequent SF.
-
Spectral type-Luminosity-Mass relation of
massive stars:
-
Galactic O star catalogue: http://dae45.iaa.csic.es:8080/~jmaiz/research/GOS/GOSmain.html.
Here is a list of nearby massive SFRs (< 1 kpc):
- (Arce et al., 2007prpl.conf..245A)
Review of types of outflow in SFRs:
(back to top)
- Common properties of SFR outflows:
- Mass-Velocity relation: the broken power law dM(v)/dv ~ v^-gamma, where gamma = 1~3 in the low velocity portion while it takes a steeper slope of gamma up to 10 in the high velocity portion. The turn point is usually at a velocity of 6~12 km/s. The slope of this relation seems to steepen with age of the SF.
- The mass, force and mechanical luminosity of the molecular outflows correlate with the bolometric luminosity.
- Many fairly collimated outflows show "hubble flow" feature.
- The degree of collimation (= length/width = major/minor radius) seems to decrease as the powering source evolves.
- Outflows from low mass stars
- typical sizes: 0.1~1 pc, typical jet velocity: 10~100 km/s, typical momentum rate: 10^-5 Msun/yr*km/s; typical outflow mass flux: 10^-6 Msun/yr.
- typical collimation factor (or degree) range from ~3 up to > 20. Higher jet velocity, higher degree of collimation.
- SiO trace the jets, not the shock front, which is awkward to the idea that SiO is a shock tracer. Three scenarios:
- SiO belongs to the jets;
- SiO is in a turbulent cocoon around the jets;
- SiO are produced and excited by internal shocks (internal working surface) that are propogating downstream the jets.
- Many jets show episodic ejection events, e.g., L 1157, HH300, L1448, HH111, etc.
- Several jets show precession, e.g., Cep E.
- Quadrupolar outflows are also found and could be bipolar outflows from two independent YSOs, e.g., HH288, L 723.
- There seems to be a time evolution trend from highly collimated jets to wider openning angle outflows.
- Good examples: HH211, HH212, IRAS 04166+2706.
- Outflows from high mass stars
- typical mass outflow rate: 10^-5 ~ a few 10^-3 Msun/yr; typical momentum rate: 10^-4 ~ 10^-2 Msun/yr * km/s; typical mechanical luminosity: 0.1 ~ 100 Lsun.
- Outflows from early-B or late O stars show high collimation factors (> 5) . Stars brigher than 10^4 Lsun show wide outflow angle of 90 deg. The entrained molecular outflow could have even larger opening angle.
- (2.2.1....)
|
|
Observations of SFRs
(back to top)
- General observation topics (back to top)
-
(Larson, 1981MNRAS.194..809L)
He anaylized a sample of molecular clouds and showed that the internal
velocity dispersion of each region is well correlated with its size
and mass in approximately power-law form: ,
.The velocity
dispersions should mainly originate from turbulence, instead of stellar
dynamical processes such as stellar winds, supernova explosion or H
II region expansion, because otherwise the the largest velocity dispersion
would appear on smallest scales, contrary to the observed trend.
(figs: )
- (Bachiller et al., 1990A&A...231..174B)
They identified two superposed outflows in L1448 and found symmetrically distributed bullets aligned along the outflow axis in CO maps. They also found braking effects in the velocity of these CO bullets.
(fig: left -- CO bullets at the extreme velocities; right -- P-V diagram showing braking effects of CO bullets.)
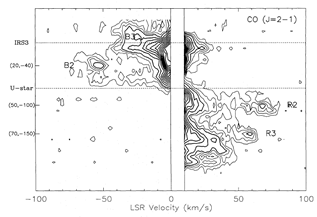
- (Bachiller et al., 1991A&A...243L..21B)
They mapped the SiO 2-1, 3-2 and 5-4 lines in the extremely high velocity outflow source L1448 using IRAM 30m. They found that SiO emission appears in the shocked gas around the bipolar outflows and around the central star, which indicates that the SiO is produced by destroying dust grains in the shock. The SiO will be depleted on to dust grains soon when the shocked gas cools down.
(fig: SiO spectra at different off-sets)
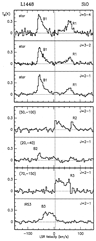
- (Martin-pintado et al., 1992A&A...254..315M)
From the IRAM 30m observation of millimeter lines from some molecules and multiple transitions of SiO in several molecular outflow sources L1448, B1, NGC 2071, and Cep A, they found that the broad SiO line profiles are distinct from other species, indicating two components in the star: quiescent gas characterized by narrow lines and shocked gas characterized by broad SiO lines. The SiO abundance was found to be greatly enhanced (by up to 10^6) in high velocity shocked gas, indicating the release of Si from the partially destroyed grains in the shocked regions.
(figs: Left to right -- Spectra of SiO and other species of cold clouds L1448 outflow (IRS3) and dark cloud B1, warm clouds NGC2071 and Cep A)
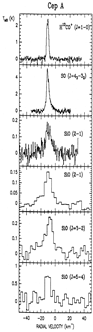
- (Dickel & Auer, 1994ApJ...437..222D)
They detected inverse P Cygni profile in HCO+ 1-0 line and blue skewed profile of HCO+ 3-2 towards the H II region,W49A North. Their radiative transfer modeling with the ability to deal with an H II region embedded inside the cloud showed that three models: free-fall collapse, homologous collapse and inside-out collapse, can reproduce the on-source line profiles equally well, while only the free-fall collapse model can account for the offset spectra. The strong continuum emission from the H II region, if hotter than the gas, can provide a background at the line frequencies to produce absorption like feature in the line profiles, although it's absorption can also weaken a little the line emission from the farther side. Different convergence acceleration techniques for the radiative transfer calculation: Approximate Lambda Operator (ALO, Olson, Auer, and Bucher, 1986) and orthogonal vector (ORTHDX, Auer, 1991) are compared.
- (Turner, 1995ApJ...449..635T)
A survey of HCO+ and N2H+ in 11 cirrus cloud cores and 28 translucent objects showed that HCO+ 1-0 was seen in all objects while 3-2 line was seen only in a few localized regions. HCO+ abundance is higher in optically thin clouds than in denser clouds, in accord with the major photo-chemistry path of the HCO+ formation. N2H+ was detected only in two densest objects, because it can be formed only by dense-cloud processes.
- (Mardones et al., 1997ApJ...489..719M)
They observed 47 candidate red protostars (some class 1 objects with IR Tbol < 200K and some class 0 objects with IR Tbol < 70K) in two optically thick lines H2CO 2(1,2)-1(1,1) and CS 2-1 and one optically thin line N2H+ 1-0 using IRAM 30m, SEST 15m and Haystack 37m radio telescopes. They found asymmetric line profiles in both optically thick lines towards most of the sources. This statistic feature can not be explained by outflow or rotation, but only by infall motions. They also found that class 0 objects have higher degree of blue profile asymmetry than class 1 objects, indicating the differences in physical environments of them. They identified 15 spectroscopic infall candidates and most of them have mainly turbulent internal mostion, rather than thermal mostion. (Q: their profile asymmetry is normalized by line width of the optically N2H+ line. However, the latter is related to turbulent motion which could be stronger in more massive objects. Thus the statistics could be biased.)
- (Motte et al., 1998A&A...336..150M)
Facts: Extended 1.3mm continuum mosaicing of rho Ophiuchi central region using the IRAM 30-m telescope with the MPIfR 19-channel bolometer array. It covers ~480 arcmin^2, = ~ 1 pc^2 at a distance of 160 pc. It is sensitive down to Ncol(H2) ~ 10^22 cm^-2. Star forming dense core and ambient clouds are mapped simultaneously for the first time. At least 58 starless cores (no IR, radio counterpart) are identfied. For cloud cores, the dN/dm ~ m^-1.5 below m=0.5 Msun, and ~ m^-2.5 above this threshold.
Opinions: The rho Oph clouds are highly structured. The dN/dm function is similar to IMF of stars, and thus the cloud cores could be the direct progenitors of individual stars and that the fragmentation of clouds play an important role in IMF. Alignment of YSOs and starless cores indicates triggered star formation by external slow shocks from young stars. They give a formula to convert dust continuum flux into H2 column density:

or
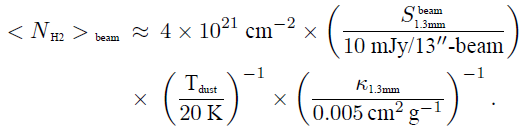
where Tdust usually =10-20K or even as high as 30K in warm clouds, kappa1.3mm = 0.02, 0.01, 0.005 cm^2g^-1 for circumstellar disk around class II YSOs, circumstellar envelope around class I and 0 YSOs, and pre-stellar dense clumps/cores, respectively. Omega_beam is the beam size, B1.3(Tdust) is the Plank function. This formula can be rearranged to estimate the total H2 mass of the cloud: M(H2) = S^beam_1.3mm * D^2 /kappa1.3mm/B_1.3(Tdust) ~ 1.734x10^-3 Msun * S(mJy)*D(kpc)^2*lambda(mm)^2/kappa(cm^2/g)/T(K), where D is distance.
- By reviewing previous studies of IMF, he summarized that Galactic-field IMF appears to be remarkedly universal, with the exception
in the substellar mass regime. Uncertainties
in the IMF mainly lie in unresolved multiple systems
and uncertainties in stellar models with rotation
and those of very young stars with age < 1 Myr.
The alpha of massive IMF could be close to the
Scalo's value of 2.7, rather than the Salpeter
value of 2.35. (from Kroupa, 2002Sci...295...82K)
- (from Gao & Solomon, 2004ApJS..152...63G)
Facts:They systematically observed HCN 1-0 line toward a sample of 53 galaxies, using IRAM 30m, NRAO12m and FCRAO 14m. The presented HCN and CO spectral plots and line parameters.The L_HCN has worst correlation with 12um luminosity than 25, 60 and 100um.
Opinions: HCN is predominantly excited by collision with H2, but not IR light. It means HCN can be used as a good tracer of dense gas and L(HCN)/L(CO) can represent well the fraction of dense gas.
- (from Gao & Solomon, 2004ApJ...606..271G)
Facts:They present the analysis of HCN, CO and far-IR luminosities of 65 infrared galaxies. They find a tight linear relation: L(IR)/L(HCN)=900 Lsun / (K km s^-1 pc^2) that is correct over 3 orders of magnitude of L_IR. However, the L(IR)~L(CO) relationship is non-linear. The star formation rate per unit dense gas mass, L(IR)/Mdense = 90 Lsun/Msun is independent of galaxy luminosity and thus eccentially the same for all galaxies, including ULIRGs. L(HCN)/L(CO) > 0.06 for all LIGs or ULIRGs and =0.02-0.05 for normal spiral galaxies.
Opinions: It is inferred that the star formation law in terms of dense molecular gas content has a power-law
index of 1.0. The star formation is the main power source in all kinds of galaxies, including the very luminous ones, ULIRGs (without the need of AGN contribution to explain their high liminosity). It is indicated that star formation rate (indicated by L(IR)) dependes more on the amount of dense gas (traced by L(HCN)) than on the total amount of gas (traced by L(CO)). The HCN global luminosity may be used as an
indicator of the star formation rate in high-redshift objects including hyperluminous galaxies. The percentage of dense gas, L(HCN)/L(CO), is a powerful starburst indicator.
(figs: 1-- L(IR)~L(HCN) relation; 2-- L(IR)~L(CO) relation (solid line fit points with L(IR)<10^11Lsun); 3-- L(HCN)~L(CO) relation; 4-- L(IR) ~ dense gas fraction relation; 5-- relation between SF rate per unit mass L(IR)/L(CO) and dense gas fraction L(HCN)/L(CO).)
- (Wu et al., 2004A&A...426..503W)
Facts: List of outflow sources: They collected all 391 known outflow sources from literature before Feb. 28 of 2003. Among them, 139 are high mass objects (judged from source mass or outflow mass). 90% of low mass outflows are associated with HH objects. 61% of high mass outflows are associated with H2O masers. 12% of the whole sample show signitures of infall or collapse.
Opinions: It is the first time to find a correlation between the outflow mass and the luminosity of the driving source. The outflows in massive objects can be two orders of magnitude more massive than in low mass stars but less collimated than in the latter.
- (Wu et al., 2005ApJ...635L.173W)
Facts: They find that bright Galactic dense cores (L_IR > 10^4.5 Lsun) show the same L_HCN-L_IR linear correlation as in nearby spiral and distant starburst galaxies: log(L_IR) = 1.01log(L_HCN)+2.83. However, fainter Galactic dense cores (L_IR < 10^4.5 Lsun) seem to deviate from this linear relation (becomes superlinear).
Opionions: They interpret this phenomenon by a characteristic dense core unit density beyond which the star forming clouds do not built up denser cores but make more core units with the same density.
(figs: left -- L(IR)-L(HCN) relation; right -- the star formation efficienc: L(IR)/L(HCN) ratio.)
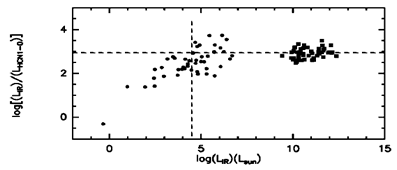
- (Beuther et al., 2005ApJ__628___800B)
Facts: They mapped the MYSO IRAS 18089-1732 in 850um band using the SMA. The continuum map showed a single cloud clump, instead of multiple cloud cores, even at so high spatial resolution. The deteced line series of CH3CN are optically thick, while only HCOOCH3 line series appear optically thin. Velocity gradient perpendicular to SiO outflow was seen only in the channel maps of HCOOCH3 lines.
Opinion: The simgle clump in the continuum map indicates that the massive cloud is still not fragmented. The asymmetric shape of the contimuum map also indicates that the morphology of the dense cloud core could be highly irregular and thus radius density profile analysis should be treated with caution. The perpendicular velocity gradient in HCOOCH3 map could be a massive rotating disk (but HCOOCH3 has two lines blended). When CH3CN is optically thick, they do not know which molecule is a safe tracer of massive disk. HCOOCH3 line series are usually very weak.
CH3CN line series are still good thermometer to determine the gas temperature however (using XCLASS). New collision rates of CH3OH is not available for radiative transfer. They discussed that there could be various ways of massive star formation, regarding when or whether the massive cloud will split into smaller clumps.
-
(Smith et al., 2006ApJ...645.1264S)
Facts: They Analysed the nature of the green color of the bipolar outflows from the massive star forming cluster in DR21 using Spitzer IRAC maps, ISO spectra and other infrared spectrometer observations. They find that all smaller regions of the green outflow show similar pixle color distribution (in a strip region) on the [3.6-4.5] -[5.8-8.0] color-color diagram (see below), while the background pixles all appear near color values of [3.6-4.5] = 0.2 and [5.8-8.0] = 1.8. The ISO spectra showed weak continuum emission in the outflow region. The previously proposed Br_alpha and CO 1-0 P(8) emission lines were not seen in the ISO spectra.
Opinion: Shock model and UV excitation models indicate that the contribution of H2 emission to the 4 IRAC bands main constitutes a large number of crowded weak lines that are not detectable in the ISO spectra. From the modeling, they find that when the shock velocity is higher and the pre-shock density is lower, the brightness distribution among different H2 lines are more homogeneous and thus the contribution of the crowded weaker H2 lines to the 3.6 and 4.5 um bands are stronger and thus produce greener structures in the IRAC maps. A combination of a C-shock with pre-shock density of 10^3~10^4 cm^-3 with a UV excitation in a region of similar density and a UV field of g=4 can explain the observed green structure in DR21. The weak continuum emission in the outflow indicates that there is little dust or PAH there. Multiple colors in each small region indicates that the flow is composed of complex shock velocity structures.
(fig: IRAC color-color diagram of each pixel.)
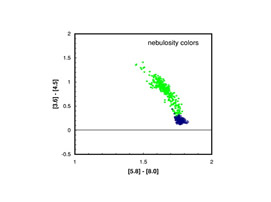
- (Churchwell et al., 2006ApJ...649..759C)
Facts: They identified 322 partial and rings that resemble 3D bubbles from the Spitzer GLIMPSE I survey. The bubbles are small, with most angular sizes between 1'~3', more than 90% sizes < 10', 88% sized < 4'. The thickness of the bubbles are usually 0.2~0.4 of the radius. They found on average >1.5 bubbles per square degree in the GLIMPSE I region (10deg<|l|<65deg on the Galactic disk) and ~ 5 bubbles per square degree in the GLIMPSE II region (|l|<10deg)! 1/4 of the bubbles coincide with known radio HII regions, 13% bubbles harbor known star clusters, only 3 bubbles are identified as known SNRs, no bubble is associated with known PNe or W-R stars. The bubbles are highly eccentric, with eccentricities peak around 0.6~0.7 and 65% of them between 0.55~0.85.
Opinion: The bubbles are mainly formed by hot stars in massive SFRs. Perhaps 1/4 are produced O-B3 stars, while the other 3/4 are produced by B4-B9 stars. The spatial distribution of the bubbles is similar to that of O, B stars.
Online material: Four-color (3.6um: blue; 4.5um: green; 5.8um: orange; 8.0um: red) maps can be find in online archive: http://www.astro.wisc.edu/glimpse/bubbles/.
- They used the SEQUOIA multipixel array on the FCRAO
14m telescope to complete a new 13CO 1-0 survey
to the Galactic Ring (l=18~55.7deg, b=-1~+1deg,
area=75.4 deg^2). The beam size is 46",
the angular sampling is 22", the velocity
resolution is 0.21 km/s, the typical rms
= 130mK. It has high spatial resolution and
better rms than previous surveys. The survey comprises 1,993,522
spectra that is available online: http://www.bu.edu/galacticring.
(from Jackson et al., 2006ApJS..163..145J)
(figs: left -- intensity
map integrated over velocity (K.km/s); middle and right --
velocity channel maps)
- (Churchwell et al., 2007ApJ...670..428C)
Facts: They identified 269 MIR bubbles that resemble 3D bubbles from the Spitzer GLIMPSE II survey (|l|<10deg) to the Galactic center region. Fewer bubbles were found near the GC due to strong background PAH emission and confusion and perhaps opacity. They found on average >1.5 bubbles per square degree in the GLIMPSE I region (10deg<|l|<65deg on the Galactic disk) and ~ 5 bubbles per square degree in the GLIMPSE II region (|l|<10deg)! Fewer bublles are found to associate with H II regions in GLIMPSE II region than in GLIMPSE I region. The bubbles are highly eccentric, with eccentricities typically around 0.6~0.8 and >50% show blowouts. At least 12% bubbles show the signature of triggered SF.
Opinion: The bubbles are are younger than in the GLIMPSE I regions.
Online material: Four-color (3.6um: blue; 4.5um: green; 5.8um: orange; 8.0um: red) maps can be find in online archive: http://www.astro.wisc.edu/glimpse/bubbles/.
-
(Robitaille et al., 2008AJ....136.2413R)
They present a highly reliable flux-limited census of 18949 point sources in the Galactic midplane that are
selected from Spritzer GLIMPSE I and II survey
(274 deg^2) with intrinsic red middle IR colors ([4.5]-[8.0]>=1).
About 50-70% of them are YSOs, 30-50% are AGB stars, 2-3% are PNe or background galaxies. 1004 red sources in the GLIMPS II region (mostly Mira
type AGB stars) show significant
(>=0.3mag) variability at 4.5 and 8 um but with constant color [4.5-8.0]
(incomplete due to 4.5um saturation and only two epochs). This is to date
the largest uniform census of AGB stars and high- and intermediate-mass YSOs
in the Galaxy. A machine readable version of the catalog is here.
- (Guan, Wu & Ju, 2008MNRAS.391..869G)
They mapped 12CO, 13CO and C18O 1-0 towards 21 SFRs using Delingha 13.7m
telescope. They resolved 53 13CO cores with 22
of which are starless. The 13CO core mass
ranges from 3.4~4.6x10^4 Msun (massive SFRs).
They used the optically thick line12CO 1-0 to derive the excitation
temperature Tex of 13CO, and then derive the opacity
of 13CO 1-0 by
.
Then they estimate the 13CO column density by
.
To derive mass of the CO gas, they assumed canonical abundances
N(12CO)/N(H2) = 10^-4 and N(12CO)/N(13CO) = N(12C)/N(13C)=89 (solar). The
radius R of the 13CO cloud core is known from their mapping observations,
then the mass can be calculated via

where mu_g=1.36 is the mean atomic weight of CO. By assuming the core is
gravitationally bound isothermal sphere with uniform density distribution, virial mass can be estimated by
(r.f., Ungerechts
et al., 2000, ApJ, 537,
221)
-
(Klaassen & Wilson, 2008ApJ...684.1273K)
They mapped SiO 8-7, HCO+
and H13CO+ 4-3 lines of 7 SFRs using HARP-B on
the JCMT. Four of them show infall signatures in their HCO+ profiles and the
infalling mass was found to be smaller than outflowing mass which indicates mass entrainment effects in the outflow.
- (Chambers et al., 2009ApJS..181..360C)
They studied a 190 cores in a sample of IRDCs. Classified them into active and quiescent cores according to the presence of "green fuzzy" and 24um point source. They found the active cores have smaller size.
- (Menshchikov et al., 2010A&A...518L.103M)
Facts: They analyzed the Herschel PACS and SPIRE images of Aquila Rift and part of the Polaris Flare regions.
Results: They extracted many starless cores from the images (541 in Aquila rift and 302 in Polaris). The starless cores are embedded in long and very narrow filament structures which are ~35" wide in Aquila Rift and ~59" wide in Polaris (~ 9000 AU in both regions). It indicates that the starless cores formed from fragmentation of filamentary clouds.
- (Andre et al., 2010A&A...518L.102A)
Facts: They analyzed the 70-500 um continuum images from the Herschel Gould Belt Survey project toward the Aquila Rift and part of the Polaris Flare regions.
Results: They extracted 350-500 prestellar cores, 45-60 Class 0 protostars, and ~300 unbound starless cores in the Aquila Rift region, while not protostars are found in the Polaris field. They confirm with much better statistics that the prestellar core mass function (CMF) resemble the IMF. They thus propose that filaments should form first and then cloud cores form from the filaments subsequently.
- Observation of low mass SFRs/YSOs (back to top)
- (Hogerheijde et al., 1997ApJ...489..293H)
Mapping of HCO+ and H13CO+ lines towards 9 low mass YSOs reveals 2/3 of them with compact disk only (point continuum sources) and 1/3 of them with envelope (extended continuum sources). HCO+ 1-0 line is found to trace surrounding clouds, while 3-2 and 4-3 lines trace the central source better. HCO+ is a better tracer of embedded YSOs than 1.1um continum and CS because the continum can originate from compact disk and CS traces surrounding clouds. A abundance of [HCO+]/[H2]=1.2(+-0.4) x 10^-8 was derived from model fitting. The intT_hco+/Lbol is a good tracer of evolutionary stages of the low mass YSOs.
- (Hogerheijde et al., 2000ApJ...534..880H)
They observed the submm continuum and 13CO, C18O, HCO+ and H13CO+ lines towards four embedded YSOs, L1489 IRS, L1535 IRS, L1527 IRS,
and TMC 1. They revealed ~2000AU elongated structures embedded in extended envelopes. The HCO+ lines from most of the sources can be well reproduced with inside-out collapse model or equally well with radial power-law of index p=1~2, except L1489 IRS which can be best interpreted by a rotation disk of the same radius of 2000AU. The CO lines can not be reproduced to the same degree, perhaps due to free-out of CO onto dust (depletion) when the gas temperature T<20 K, the sublimation temperature of CO.
- (Cazaux et al., 2003ApJ...593L..51C)
Facts: They performed a sensitive mini survey to search for organic molecules in the low mass protostar IRAS 16293-2422. They detected all characteristic organic molecular species in high mass hot cores. However, they find that the CH3OCHO/CH3OH abundance ratio is close to unity in this low mass hot core, which is about 10 times higher than in typical high mass hot cores, whilst the N-bearing species show similar abundance ratios as high mass hot cores.
Conclusions: Low mass protostars also have associated hot cores with complex chemistry. The difference in the ice composition could be the reason for the overabundance of O-bearing species, in agreement with the 2 order of magnitude higher deuteration degree in low mass hot cores than in high mass hot cores. Other possible factors are gas temperature and timescales.
- Observation of massive SFRs/YSOs (back to top)
-
---Formation of massive stars via single star
accretion--- (back to top)
-
Radiation and hydrodynamic model calculatiions show that the standard Galactic dust-to-gas ratio should be reduced by
a factor of 4 or more to allow accretion of gas onto a very massive star. Intermediate sized grains (0.05-0.25um) must be missing to allow inflow of
gas in the presence of very high radiation pressure. The accretion rate must be ~ 10^3 Msun/yr or more to
pruduce strong enough ram pression to overcome the decceleration of the
radiation pressure. Thus the formation of an isolated massive star
requires special preconditioning of the interstellar medium. (from
Wolfire & Cassinelli, 1987ApJ...319..850W)
-
Typical star formation time scale is t*f ~ 10^5
yr. It is only weakly dependent on final stellar mass, and thus massive and low-mass stars can form simultaneously in a
cluster. If the accretion of matter is in the form of disc and outflow, the accretion rates are
sufficient to overcome the radiation pressure from a 100 Msun star. (from Mckee & Tan, 2002Natur.416...59M)
-
They mapped CO 2-1 outflows in 69
luminous IRAS point sources. 60 are massive SFRs (L_IRAS=10^3~10^5 Lsun, associated with NH3 dense core). 39 regions show high velocity wing of CO and patially
confined outflow. Detection rate of outflow is 90%.
Many outflows contain mass more than 10 Msun and momenta more than
several hundred Msun*km/s, indicating outflow from massive
YSOs. Massive stars may form from accretion
of a signle star. (from Zhang et al., 2001ApJ...552L.167Z)
-
They present a flattened disk-like structure around a 15 Msun protostar in Cepheus A region, based on the continuum emission
from dust and line emission from molecular gas. The disk has a radius of
330 AU, and a mass of 1~8 Msun. It is oriented perpendicular to, and spatially coincident with, the central embedded powerful radio jet, just as the case of low mass star formation. (from Patel et al., 2005Natur.437..109P)
-
IR luminosity of a sample of 69 IRAS point sources (candidate massive
SFRs) is 10^2~10^5 Lsun. CO 2-1 shows that molecular outflow is ubiquitous in these massive SFRs.
Mass of the outflows are tens of Msun,
typical dynamical timescale is 10^ 4 yr,
typical energy is 10^46 ergs, comparable to
the turbulent energy of the core. Nearly half of the outflows show bipolar lobes. The maximum outflow mass loss rate is 10^-4
Msun/yr, indicating that the accretion rate is of the same order. (from Zhang et al., 2005ApJ...625..864Z)
-
They detected spherical infall of
molecular gas around UCH II region G10.6-0.4 through mapping of NH3 (3,3) line and 23 GHz continuum. The infall doesn't flatten to
disk at radii as small as 0.03 pc, indicating that the molecular infall
has pricked through the H II region and developed into ionized infall inside the H II region, instead of
halting at the outer edge of the expanding H II region. (from
Sollins et al., 2005ApJ...624L..49S)
-
LVA observation of NH3 (3,3) line and 23 GHz continuum in UCH II region G10.6-0.4 revealed a flattened
rotating molecular accretion flow, but no accretion disk was found,
which suggesting a different cluster formation model of massive stars in
which massive stars form in cluster and accretion
flow does not develop accretion disk. (from Sollins & Ho, 2005ApJ...630..987S)
-
They mapped CO 2-1 in 12 massive SFRs. Together with previous
observations, detection rate of outflow is 88%.
The frequency distribution of outflow collimation factor show no
difference between massive and low mass SFRs, indicating that massive stars also form through accretion. (from Kim & Kurtz, 2006ApJ...643..978K)
-
3-D AMR modeling of the massive star formation from the gravitational
collapse of massive, magnetized molecular cloud
cores showes that massive stars can
be formed more quickly than throught before, with accretion
rate > 10^-3 Msun/yr. The accretion rate is 20-100 c^3/G, larger than the value of 0.98C^3/G
predicted by self-similar collapse solutions (c is sound speed, G is
gravitational constant). Bipolar outflows produce cavities to release radiation pressure.
Disk-threaded magnetic fields and the
appearance of bars with spiral arms help extract angular
momentum and thus increase the accretion rate. (from Banerjee
& Pudritz, 2007ApJ...660..479B)
-
JCMT single point observation of SiO,
HCO+ and H13CO+ towards 23 massive SFRs revealed detection of SiO in 14 sources, SO2 in 17 sources and HCO+
in all 23 sources. Mass infall rates are in the range from 10^-2 to 2x10^-5 Msun/yr. SiO broad emission only appear in young outflows.
HCO+ is a tracer of infall motion. Seven sources have both SiO emission
and HCO+ infall signature detected, indicating they are objects with
both outflow and infall. They suggested
that both ionized accretion and halted accretion
may be important in evolution stages beyond H II formation. They
used the formula to calculate the column density of the optically thin
line H13CO+:
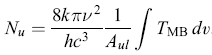
(from Klaassen & Wilson, 2007ApJ...663.1092K)
- They mapped SiO 2-1, H13CO+ 1-0, NH3 (J,K)=(1,1)(2,2) lines and the 1.3mm and 3.4mm continuum towards two massive SFRs IRAS 18264-1152 and IRAS 23151+5912. For IRAS 18264-1152, they proposed precessing jets to explain the observed kinematics. For IRAS 23151+5912, They suggested entrainment of the gas by an underlying wide-angle wind to explain the observed morphology. They also confirmed the broken power law of M-V relations in SFRs. (from Qiu et al., 2007ApJ...654..361Q)
(figs:
upper row: IRAS 18264: left -- SiO channel maps; middel -- H13CO+ channel maps; right -- SiO spectra of both stars;
lower row: IRAS 23151: left -- SiO channel maps; middel -- H13CO+ channel maps; right -- broken power law mass-velocity relations of both stars.)
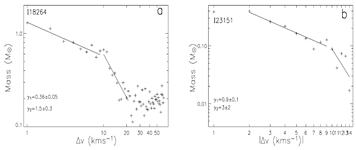
- They mapped the CO 3-2, HCN 4-3 and SiO 5-4 towards the luminous YSO IRAS 20126+4104 with the SMA at a resolution of 1'-2". They found three kinematic components in the bipolar outflows: a) a compact young (age of ~120yr) bipolar outflow near the central star; b) a more extended collimated bipolar outflow traced by SiO line; c) the most extended S-shaped outflow traced by CO 3-2. An episodic precessing jet is proposed to explain all three components. The SiO abundance is estimated to be (1-5)x10^-8 in the outflow lobes. A hubble flow was found in the red-shifted side with high velocity at the tip, (posing a question why it did not decelerate?) (from Su et al., 2007ApJ...671..571S)
(figs: upper row (CO); lower row (HCN & SiO): left -- map; right -- P-V diagrams (PA=120deg for HCN and SiO))
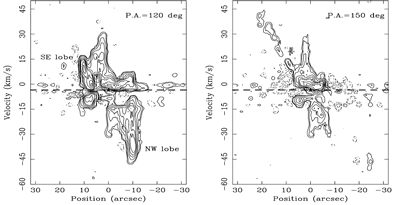
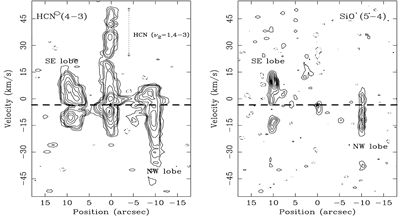
- (Qiu et al., 2009ApJ...696...66Q)
By SMA observation of 12CO, 13CO 2-1 and 1.3mm continuum of G240.31+0.07 with high spatial resolution, they find it to be a massive YSO showing broad angle outflow in a cluster environment.
- (Kraus et al., 2010Nature_466__339K)
Facts: Using IR interfereometry technique, they discovered an hot elongated disk structure around a massive YSO of ~ 20Msun. The projected linear size of the disk is 13x19 AU, indicating an inclination angle of ~45degree. The determined temperature gradient in the disk and the inner dust free region of less than 9.5 AU look similar as a disk around a low mass YSO. They also recognized a outflow and some bow shocks in the direction perpendicular to the disk.
Opinions: Disk accretion is possible in a forming massive star of 20Msun.
-
---Formation of massive stars via collision of stars--- (back to top)
- They present a model for the formation of
massive (M>~10 Msolar) stars through accretion-induced
collisions in the cores of embedded dense stellar
clusters. The stars accrete zero-momentum gas to lose angular
momentum and fall into the center of the cluster to trigger stellar
collisions to form very massive stars. This explain why massive stars
usually appear in the center of clusters and in close binary systems.
Once a massive star forms, the high lominosity will expel the
zero-momentum gas from the cluster and thus stop the contraction of the
cluster and halt further collisions. (my question:
does the gas really have zero-momentum?) (from Bonnell et al., 1998MNRAS.298...93B)
-
They proposed that low mass close binaries at the center of binary
will grow up to massive close binaries at the cluster center. These close binaries are usuall highly eccentric and
easy to merge to form a massive star of
30-50 Msun, without the problem of radiation impeding accretion. Such
merger mechanis requires only 1% of stellar density at the cluster
center than the direct stellar collision mechanism, say ~ 10^6
stars/pc^3. (from Bonnell & Bate, 2005MNRAS.362..915B)
- ---Formation of massive stars via competitive accretion
in a small stellar cluster--- (back to top)
-
3-D SPH dynamical modelling of a small cluster containing 3-10 stars showes that
the accretion of member stars are not uniform. Several stars in the center of the cluster accrete more gas and thus form more massive stars. This is called competitive accretion. It occurs because at the
cluster center, the stars can take advantage of the gravitational
potential of the whole cluster to draw more gas to their vicinity to
achieve more efficient accretion. The membership in a binary or multiple system also has higher accretion rate due to stronger collective gravitational
potential. The competitive accretion take a role in determing the stellar mass spectrum. (from Bonnell et al., 1997MNRAS.285..201B)
-
They argued that the competitive accretion is the most possible formation mechanism of massive
stars. Stars in the center of a small cluster can accrete distributed gas via the funnelling
flow in to the cluster center, and thus acquire more mass than an
isolated star. If the local properties of the
cloud at the center of a cluster are used, the competitive
accretion is promising. With 100 times
higher density (10^-17 g/cm3), 5 times lower velocity dispersion (0.4
km/s, due to velocity-sizescale property of turbulence, V~R^1/2), 5
times higher stellar mass (0.5 Msun), they derive 10^5 times larger
accretion rate (10^-4 Msun/yr) than using the average properties of the
natal turbulent cloud. (from Bonnell & Bate, 2006MNRAS.370..488B)
-
We demonstrate that the mass of the most massive
star in a cluster correlates non-trivially
with the cluster mass. A cluster forms by more massive
stars being consecutively added until the resulting feedback
energy suffices to revert cloud contraction and stops further star
formation. The IMF will be different for a
collection of smaller clusters and a large cluster of the same total
mass. (from Weidner & Kroupa, 2006MNRAS.365.1333W)
- ---Other observations of massive SFRs---
(back to top)
- (Caselli & Myers, 1995ApJ...446..665C)
Facts: They observed 13CO and C18O 1-0 lines and maps of massive cloud cores in Orion A (L1641) and B (L1630) to study the line width-radius relation of the cloud cores. They used a thermal + non-thermal motion model (TNT model) to help interpret the data.
Conclusions:
(1)
The massive cloud cores have a shallower non-thermal velocity (dV_NT) and cloud ratius (R) relation dV_NT ~ R^q with q=0.21+-0.03 which is smaller than that of low mass cloud cores (q=0.53+-0.07).
(2) The existance of a forming star or a small group of stars do not affect the line width much.
(3) The existance of protostar cluster seems to enhance the amount of non-thermal motion in the cloud cores.
- (van der Tak et al., 2000A&A...361..327V)
Observations of CH3OH and H2CO in 13 massive
SFRs showed that Trot = 30-200K for CH3OH and Trot = 60-90K for H2CO. The CH3OH
line width is only 3-5 km/s, except in two
sources where the line shape reveals outflows.
Chemistry modeling shows that the abundance of CH3OH
has a jump-up in the inner core above 100K, while H2CO show flat abundance
distribution in the SF cores.
-
(van der Tak et al., 2003A&A...412..133V)
Observation showed that 10-15% of SO and SO2 line profiles show infall or outflow signatures
in massive SFRs. Trot =
25K for H2S, 50K for SO, H2CS,
NS and HCS+, and 100 K for OCS and
SO2, indicating that most molecules trace the outer part
(T<100K) while OCS and SO2 trace the inner part of
the envelope. OCS has the highest abundance and
thus is possibly the major reservior of sulphur.
H2S and SO lines come from the outer part of the clouds and thus can not
trace the heating of the star forming clouds.
- (Wu & Evans, 2003ApJ...592L..79W)
They detected blue profiles of HCN 3-2 line in 12 of 28 massive SFRs, while only 6 of them show red profile. This high percentage of blue profile indicates that HCN 3-2 could be a good infall tracer in massive SFRs.
-
(Wu et al., 2005AJ....129..330W)
They mapped CO 2-1 towards 11 massive SFRs using the 8-beam array of ARO 12m telescope. Bipolar
outflows were identified in 6 sources, while multiple
velocity components of the CO line profiles hindered identification
of outflows in the remaining 5 sources. Mass of the outflows ranges from a few to 60 Msun, and the momentum amounts to a few hundreds Msun*km/s. Both are much larger than
in low mass formation regions and indicate energy source other than
radiation. The average dynamical timescale of the outflows is 2x10^4 yr. They also found that the
outflow luminosity and mechanical force correlate with the luminosity of the
star.
- (Purcell et al., 2006MNRAS.367..553P)
They performed a 3mm spectral line survey towards 83 CH3OH maser selected massive SFRs. CH3CN 5-4 and/or 6-5 were detected towards 70% of the sample, indicating Tex = 28~166 K. Although brighter CH3CN lines were detected more in UCH II regions, some detections of them in non-UCH II sources hints that CH3CN is already excited before the UCH II stage. HCO+ 1-0 was detected in 99% of the sample, however, equal number of blue and red profiles were found in the whole sample. 12 dark clouds in the sample show 90% detection of CH3CN and 7/12 detection of HCO+, and a strong trend of more blue profiles indicates infall motion in them.
-
(Ellingson, 2006ApJ...638..241E)
The tight association of 6.7 GHz CH3OH masers with
very red Spitzer GLIMPSE point sources and IRDCs indicates that these masers arise from massive SFRs. Comparison of the
GLIMPSE point sources associated with different masers suggests that: Class I methanol masers trace
generally earlier evolutionalry phases than Class
II methanol masers, while OH masers trace an even later
evolutionary stage.
-
(Rathborne et al., 2006ApJ...641..389R)
Infrared dark clouds (IRDCs) are dense
molecular clouds seen as extinction features against the bright mid-infrared
Galactic background. Millimeter continuum maps using IRAM 30m toward 38 IRDCs reveal extended cold
dust emission to be associated with each of the IRDCs. IRDCs range in
morphology from filamentary to compact and have masses of 120
to 16,000 Msolar, with a median mass of ~940 Msolar. Each IRDC contains at least
one compact (<=0.5 pc) dust core and most show multiple cores. We find 140 cold millimeter
cores unassociated with MSX 8 μm emission. The core masses range from 10 to 2100 Msolar, with a median mass of ~120 Msolar. The slope of the IRDC core
mass spectrum (α ~ 2.1 +/- 0.4) is similar
to that of the stellar IMF. Assuming that each
core will form a single star, the majority of the cores will form OB stars.
IRDC cores have similar sizes, masses, and densities as hot cores that are associated with individual, young high-mass
stars, but they are much colder. We therefore suggest that IRDC represent an earlier evolutionary phase in high-mass
star formation. In addition, because IRDCs contain many compact cores
and have the same sizes and masses as molecular clumps associated with young clusters, we suggest that IRDCs
are the cold precursors to star clusters.
-
(Rathborne et al., 2007ApJ...662.1082R)
Using the PdBI, they detected 12 bright
compact condensitions (continuum emission) in
four high mass (200-1800Msun) IR dark clouds (IRDCs).
Two of them show multiple systems, one showes two components and the last show a single component. They are in the early stage of
MYSOs in clusters.
-
(Cyganowski et al., 2008AJ....136.2391C)
Using the Spitzer GLIMPSE data, they
identified 300 extended 4.5um sources (extended
green objects, EGOs) that could be early stages of massive YSOs with outflows, because
(1) The shock tracer lines H2 v=0-0, S(9,10,11) and/or CO v=1-0 are the strongest in the 4.5um band than in the 3.6 and 8um bands..
(2)
The
majority of EGOs are also correlated with IR dark
clouds (IRDC) and CH3OH 6.7 GHz masers that are MYSO tracers as well.
(3) The GLIMPSE survey is a shallow survey, and thus the H2 emission in low mass SFRs can not be detected by the GLIMPSE survey.
(4) Only in early stage of SF when the YSO is deeply buried or not hot enough and thus do not excite large-scale PAH emission in the 8um band, 4.5um band can stand out in these EGOs.
- (Goicoechea, 2008EAS....31...73G or 2008arXiv0801.0293G)
Summary of ISO FIR spectra of the two representative massive star
formation clouds Sgr B2 and Orion KL:
---Sgr B2---
(1) Peak of dust thermal emission (Tdust ~ 30K, peaks around 100um);
(2) Atomic fine structure
lines: O I, O III, C II, N II, N III (major coolants and excellent
discriminants of PDR, HII and shock emission). Almost all of them are in
emission, except the two absorption lines [O I] 63um and [C II] 158um;
(3) Rotational lines from key light hydrides (H2O, OH, H3O+, CH2,
CH, NH3, NH2, NH, HF, HD, H2D+) and ro-vibrational
lines from non-polar molecules (C3).
Most of them are in absorption.
The FIR features mainly trace warm, low density envelope of Sgr B2
(Tk=300~500K, nH2<10^4 cm^-2) where high-J CO line is not detectable for
ISO. These regions are difficult to trace in mm regime where mainly molecular emission lines from denser region are visible. All
ground state molecular lines in Sgr B2 show broad absorption due to
foreground absorption.
---Orion KL---
FIR spectrum is dominated by emission from molecular (H2O, OH, NH3, high–J CO) and atomic species;
Hige resolution line profiles of H2O and OH
show complex behaviors: changing from pure absorption to P Cyg profile, to pure
emission, depending on line frequency, Eup and opacity. CO lines up to J=39 has been detected.
---Properties---
(1) At FIR, dust emission in
both SFRs is optically thick, thus both the
observed continuum and line emission arise from the outer layer of the
clouds;
(2) With the high critical density of the molecular lines
in FIR, the most transitions are hardly collisionally thermalized, but are radiatively thermalized by the strong dust emission
field. In contrast, at mm or longer wavelength regeme, the molecular
transitions are usually collisionally thermalized and thus the dust
emissiong and opacity can be neglected. Therefore, coupled
dust and gas radiative transfer is necessary for the FIR lines.
Furthermore, the molecular excitation becomes highly non-local
and non-LTE, which renders the LTE and LVG
simulation not applicable any more. Further complexity may arise from
the presence of velocity field and overlapping lines.
-
(Sun & Gao, 2009MNRAS.392..170S)
They observed HCO+ 1-0, CS 2-1, HNC 1-0, HCN
1-0, CO 1-0, 13CO 1-0, and C18O 1-0 towards 29 massive star forming cores using Delingha 13.7m
telescope. Inward motion was prominent in the blue profiles of HCO+, CS and
HCN lines. Different optically thick lines show different amount of blueing
of the line profiles, while HCO+ is the best infall
tracer among the three. They identified 6 strong infall candidates: G123.07-6.31, W75(OH), S235N, CEP-A, W3(OH) and NGC 7538.
-
(Beuther et al., 2009AJ....137..406B)
Combining previous SMA observations, they
characterized the chemical evolution in more
detail in four massive SFRs. The four sources
show different characteristics, which result from different gas temperatures
or chemical effects. The weaker CH3OH lines and CH3CN lines in two early high-mass protostellar
objects (HMPOs) than in the two later hot molecular cores (HMCs) indicate
that they are made and excited in later stage of SF when
the cores have energy source to heat up the gas and grains.
-
(Cunningham et al., 2009ApJ...692..943C)
Their NIR H2, radio CO and thermal IR observations of nearby massive
SFR Cep A (725 pc from us) indicate a pulsed, precessing jet that have changed its
orientation by 45 deg in roughly 10^4 years. The current jet is in accord with the
findings of a disk perpendicular to the current
orientation of the jet and with the findings of a
companion with a separation comparable to the disk size. Numerical simulation with a 15Msun star with a
circumstellar disk orbited by a companion in an inclined eccentric orbit can
explain the observation when the companion periodically approach the young
star and cause the disk precession.
-
(Fontani et al., 2009arXiv0903.0159F)
They mapped a very young cluster IRAS 05345+3157 that is composed of
intermediate and high mass prestellar core and protostars. They found the
evidance that the turbulence produced by
powerful CO outflows from two massive
protostars can influnece the evolution of
another two low mass prestellar cores.
- (Chen et al., arXiv:0903.4223v1)
They find that ~67% of EGOs (extended green objects) are associated with known class I mechanol masers within 1 arcmin, which demonstrates the physical association between class I methanol masers and outflows on a statistical basis for the first time. However, only 61 EGOs (among the total of 300) have been observed in the maser, and thus the remaining 240 EGOs form an attractive sample of outflow sources to search for class I methanol masers.
- (Embritchtinger et al., 2009A&A...496..731E)
They used multi-transitions of CO and 13CO lines from Jup=3 to Jup=13 to study the structure of massive star formation region NGC 2024 (assoicated with a known HII). They found three components: a 300K hot component at the interface between the HII region and the molecular cloud; a warm (75K) and dense (9x10^5 cm^-3) gas component, and a very cold foreground component (20K). These can be interpreted by the combination of temperature and density structures of a PDR model and the velocity field of a blister model.
- (Fontani et al., 2010A&A...517A..56F)
Facts: They surveyed Class I (44 and 95 GHz) and Class II (6.7 GHz) CH3OH masers toward 296 massive SFRs that can be divided into two groups: high and low, according to their IRAS [12-25], [25-60] colors.
Results: They detected 55 class II masers, 27 class I (44 GHz) masers, 11 class I (95 GHz) masers.
Conclusions: The detection rates of Class I and II masers are similar in both high and low sources, but the detection rates of all masers are 3 times larger in the high group of sources than in the low group. The methanol masers become progressively stronger when evolving from the early low state to the later high stage.
-
Single dish spectral survey towards massive SFRs:
Blake et al. 1987; MacDonald et al. 1996; Schilke et al. 1997; Hatchell et al. 1998; McCutcheon et al. 2000; van der Tak et al. 2000, 2003; Johnstone et al. 2003; Bisschop et al. 2007.
|
Theory and modeling of SFRs
(back to top)
-
General theories and models of SFRs (back to top)
- (Hoyle, 1953ApJ...118..513H)
He traced the steps by which an extensive cloud of density 10^-27 gm/cm3 evolves, first to galaxies, then to stars. It's indicated that galaxies tend to form in clusters and stars tend to form in early stage of galaxy history.
There also seem to be a reason why the average mass of
stars should be lower than the Sun.
- (Goldreich & Kwan, 1974ApJ...189..441G)
Radiative transfer in a homogeneous free-falling molecular cloud around HII region with LVG method.
- (Leung & Liszt, 1976ApJ...208..732L)
A radiative transfer calculation of CO is performed for static homogeneous spherical molecular clouds with microturbulence. Microturbulence is that of sizescale smaller than the photon free path length, while macroturbulence is that of sizescaleA larger than the photon free path length. Microturbulence enter the radiative transfer calculation in the same manner as thermal motion and affects the opacity of molecular lines. Macroturbulence does not affect the local radiative transfer, but broadens the emergent line profile without changing the equavolent width of the line. However, because the opacity varies much across the line profile, the turbulence could be micro in line wings but macro in the line center. The difference between systermic motion and turbulence is not easy to tell in emission lines, but can be witnessed in absorption lines, because the former shift the absorption towards one side of the line, while the latter broaden the absorption line on both sides of the line profile. A large body of CO line observations can be successfully reproduced with [CO]/[H2] ~ (1-4)x10^-5. Opacity effects in 12CO lines can bring the isotopic line ratio down to observed value of 12CO/13CO ~ 4, even if the 12C/13C ~ 40.
- (Cabrit & Bertout, 1986ApJ...307..313C)
They investigated the line profiles and P-V diagrams of simple bipolar outflow models with different radial velocity laws, inclination angles and opening angles. They also noted that the traditional methods used to deriving parameters with CO maps usually underestimate CO column density and outflow time scales due to the assumption of optically thin line.
- (Adelson & Leung, 1988MNRAS.235..349A)
They simulated the effects of rotation and radial expansion on spectral line profiles and P-V diagram. Both motions have the potential to produce similar asymmetric line profiles in optically thick lines. In the case of rotation, the telescope is needed to offset from the source center to produce the asymmetry.
-
(Myers et
al., 1996ApJ...465L.133M)
They proposed a simplified two component model of
spherical infalling clouds to expore the (self-absorption) blue profile (with double peak or red shoulder)
in SFRs. The physical quantities such as density, infall velocity, radial
position and temperature of the two homogeneous components are taken as
opacity-weighted quantites from detailed cloud model. They give a quantized
formula to estimate infall velocity Vin from
observable quantities of the blue profiles:
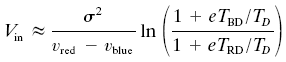
where sigma is the velocity dispersion that can be obtained from FWHM of
optically thin lines, Vred and Vblue are the velocity shifts of the blue and
red peaks w.r.t. to the systemic velocity, T_D is the brightness of the dip
(between the two peaks), while T_BD and T_RD are the brightness temperature of
the blue and red peaks respectively. This formula is only valid when Vin < sigma (no matter thermal or
nonthermal dispersion). (My comments: too simplified!
need formulation on the basis of detailed physical models.)
- (Rawlings et al. 2000MNRAS.313..461R)
Radiative transfer modeling of observed HCO+ line strengths always give an order of magnitude higher HCO+ abundance (~10^-8) than predicted by reliable chemical model (~10^-9). They proposed the formation of additional HCO+ on the wall of collimated outflows to account for the excess of HCO+. The shocks of the outflow desorb simple molecules from grains and the intenseUV radiation produced in the shock fronts can drive the photochemistry to produce much HCO+. A detailed chemistry model was presented. It also indicates that more complex model than spherical quiescent clouds is required to interpret the profile and morphology of the HCO+ lines, particularly in low mass SFRs in which collapse motion is invariably accompanied by collimated outflows.
- (Rawlings et al., 2004MNRAS.351.1054R)
They proposed a 3D radiative transfer code to reproduce the HCO+ spectral lines and maps with the photochemistry in the wall of a bipolar-outflow model. Their model can reproduce the observation of star forming core L1527.
- (Krumholz et al., 2005Natur.438..332K)
Facts: They studied the viability of the two major star formation mechanism: gravitational collapse and competitive accretion, by compare simulations with observations.
Conclusions: No competitive accretion can occur in the conditions of real star forming clouds. Thus, most stars should form by gravitational collapse.
-
(Ray et al., arXiv:astro-ph/0605597 or 2007prpl.conf..231R)
They summarized the present status of jet studies:
-
High resolution optical imaging of jets in CTTS clearly revealed the jet diameter variation which indicates that the collimation is achieved on scales of a few tens of AU (close
to the YSO) and thus rules out the hydrodynamical
focusing models.
-
The jet width is about 20-40 AU at a
projected distance of 100AU from the star.
-
The variation of jet width with distance from the source is consistent
with disk wind models of moderate or high
efficiency (Mlr_eject/Mlr_accretion > 0.03).
-
Jets show at their base an onion-like kinematic
structure, being more collimated at higher
velocities and excitations.
-
At a distance of 50-80 AU from the star, the low velocity components
gradually disappear, indicating that the jets
accelerate on scales of 50-100 AU (does it
further accelerate?). These can be explained by the magnito-centrifugal wind model.
-
Line of sight velocity asymmetry of 5-25 km/s
across the jet axis was found in CCTS DG Tau and Th 28, which
indicates that the jets are rotating. Such
rotating jets are also found in two small scale jets: HH 26 and HH 72.
The jet rotation also supports the centrifugally acceleration
mechanism.
-
The estimated angular momentum carried in the rotating jets account
for 60-100% of the angular momentum needed to be removed from the
accretion disk to allow the observed accretion rates, implying that jets
can be major agents of angular momentum dissipation.
-
Alternative models such as precessing jets or warped disk can not explain why velocity
asymmetry is so common and why the extent of asymmetry is always in the
range interpretable by disk wind model.
-
However, many puzzles remain:
- RW Aur is found by Cabrit et al., 2006 to have bipolar jets rotating in the opposite sense of the
its disk;
-
The velocity of the two bipolar jets can be different. E.g., RW
Aur has radial velocity of 190 km/s for its blue jet while only
about 100-110 km/s for its red jet.
-
Jet is ubiquitous among CTTS, but much weaker in more massive Herbig
Ae/Be stars or less massive brown dwarfs, indicating that the conditions for producing jets is optimised in CTTS.
- (Kromholz & Thompson, 2007ApJ...669..289K)
They proposed a unified theory for the two typical forms of Kennicutt-Schmidt (KS) laws: (1) SFR ~ L_IR ~ L(CO)^1.5 and (2) SFR ~ L_IR ~ L(HCN). Their theory is that, for any gas tracer, if the median cloud density is higher than the critical density of the tracer (e.g., the case of CO), the SFR is propotional to gas density that determines how much gas is available for forming stars and get another factor of the square root of gas density due to the dependence of the free-fall or dynamic time on the gas density; if the meadian cloud density is smaller than the critical denstiy of the gas tracer (e.g., the case of HCN), the observed line only sample the high density portion of the clouds and thus the cloud density in these high density portions are the same in all galaxies and only depends on the property of the molecule, then the dynamic time is the same for all clouds and thus SFR is linearly related to gas luminosity.
- (Kromholz et al., 2009ApJ...699..850K)
They propose that the local star formation rate in galaxies are controlled by three factors: (1) the interplay of radiation field and gas self-shielding that determines the amount of gas in molecular form and thus is eligible to form star; (2) the internal feedback that determines the properties of the cloud, which is independent of the galaxy properties until the inter-cloud gas pressure becomes comparable to the internal cloud pressure; (3) the turbulence caused by the feedback processes makes the star formation slow (only convert a small fraction of ~1% gas into stars per free-fall time scale). If the inter-cloud gas pressure overtakes the internal cloud pressure, the SFR starts to be controlled by the galaxy properties. They find that this transition seems to occur around H2 or total gas surface density of ~ 100 Msun/pc2. This model can explains the observed correlations between SFR and metallicity, and surface density of atomic, molecular, and total gas. For example, the SFR is correlated well with the molecular gas but poorly with atomic gas, the correlation between the SFR and the molecular gas stellar surface density is linear for clouds with surface molecular mass surface density between 5-100 Msun/pc2 but becomes superlinear for a surface density of ~ 1000 Msun/pc2.
- (Cunningham et
al., 2009ApJ...692..816C)
They simulated the interaction between jets and turbulence and found that
(1) turbulence can alter the jet morphology
while (2) the jets are able to re-energize decaying
turbulence at larger size scales , contrast to previous conclusions.
- Theories and models of low mass SFRs (back to top)
- Larson-Penston flow model...(to read) (from Larson, 1969MNRAS.145..271L)
- Larson-Penston flow model...(to read) (from Penston, 1969MNRAS.144..425P)
- Similarity solutions are obtained for
gravitational collapse of isothermal gas spheres. The collapse starts from the center of the sphere, then the collapse wave expands outwards into the outer static layer with sound speed -- inside-out. The gas swept over by the collapse wave moves inwards in free fall, because the gas beneath it has been removed. The physical structures inside the collapse wave is that of free fall gas: density rho ~ r^-3/2, collapse velocity u ~ r^-1/2, while outside of the collapse wave, the gas is still in static equilibrium (u=0, rho ~ r^-2). (It's still not clear why the collapse starts from the center of the cloud.) (from Shu, 1977ApJ...214..488S)
- He modeled radiative transfer of CS molecular lines in a 1-D low mass dense core to test the two well known protostar collapse theories: Larson-Penston flow (with the fastest collapse velocity) vs inside-out collapse (with the slowest collapse velocity). The observation and modeling support the inside-out collapse model, not the Larson-Penston flow. (from Zhou, 1992ApJ...394..204Z)
-
X wind model. (from Shu et al., 1994ApJ...429..781S and 1995ApJ...455L.155S)
-
Disk wind model. (from Pudritz et al., 2007prpl.conf..277P)
-
Chemistry modeling work: Caselli et al. 1993; Millar et al. 1997; Charnley 1997; Viti et al. 2004; Nomura & Millar 2004; Wakelam et al. 2005; Doty et al. 2002, 2006.
- Theories and models of massive SFRs (back to top)
-
(Li & Nakamura, 2006ApJ...640L.187L)
Facts: They did 3D MHD simulation of cluster formation region with turbulence.
Opinions: They concluded that the primordial (interstellar) turbulence dies away quickly and be replaced by protostellar turbulence (produced by stellar outflows). The forming cluster will form a centrally condesed pivotal state.
-
(Nakamura & Li, 2007ApJ...662..395N)
Facts: They did 3D MHD simulation of cluster formation region with turbulence.
Opinions: They concluded that the primordial (interstellar) turbulence dies away quickly and be replaced by protostellar turbulence (produced by stellar outflows). The forming cluster will form a centrally condesed pivotal state.
- (Krumholz et
al., 2009Sci...323..754K)
3D simulation showes that the radiation pressure
does not halt the gas accretion during the formation of massive
stars. Instead, the gas streams on to the disk hydrodynamically, thanks to Rayleigh-Taylor instability.
- Chemistry issues in SFRs (back to top)
- Reviews on chemistry issues:
- Chemical evolution in star forming regions: ...(from van Dishoeck & Blake, 1998ARA&A..36..317V)
- Chemistry in interstellar ND-shocks in dense clouds (no photodissociation) : They computed the chemistry in a non-dissociative shock (ND-shock) with velocity of 8 km/s. They model starts from elemental abundances H:O:C:Si:S=10^4:5:3:0.3:0.1, [C/CO]=2x10^-3, [O/O2]=10^-3, (and most other elements are atomic in the shock region, ?my guess). Substantial enhancement is predicted for H2S, SO, SiO compared to that in cold interstellar clouds. SiO is formed immediately behind the shock front through reactions with the abundant species OH through Si + OH = SiO + H. Although this reaction is slow, SiO is even harder to destroy once it is formed (SiO + C = CO + Si, SiO + OH = SiO2 + H are much slower), and thus its abundance remains as high as [SiO/H2] ~ 2.5x10^-8 to a large distance behind the shock front. SO and SO2 also form behind the shock front and remain as a constant abundance of [SO/H2] ~ 3.7x10^-8 and [SO2/H2] ~ 3.7x10^-11 to a large distance. (from Hartquist et al., 1980ApJ...236..182H)
(Tabs: left -- the shock model, with x the distance behind the shock front; middle -- the abundances of H and O containing species; right -- the abundances of minor species.)
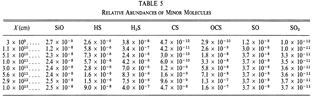
- Passage of a non-dissociative shock of 10km/s through an diffuse interstellar cloud of initial density of 100 cm^-3 induces chemistry. The pre- and post-shock column densities are listed in a table. Also given are time evolution curves of those evolving species. The enhancement of HCO+ in the postshock gas of SNR IC 443 is due to high abundance of C+ in the preshock cloud. (from Mitchell & Deveau, 1983ApJ...266..646M)
- (Mitchell, 1984ApJS...54...81M)
Effects of shocks on the molecular composition of a dense interstellar cloud (n=10^4/cc). Calculation are done for Vshock = 5~20 km/s. The shock becomes dissociative above 17 km/s. For the case of non-dissociative shock with Vshock = 5-15 km/s, CO, N2 and CO2 are little affected by the shock, while the molecules H2O, H2CO, CH2, CH3, CH4, NH3, and HCN usually attain very high postshock abundances. The synthesis of large hydrocarbon spiecies is not as efficient as in diffused clouds.
- (Mitchell, 1984ApJ...287..665M)
Effects of shocks on the sulfur chemistry of a dense interstellar cloud (n=10^4/cc) are followed for 10^5 years of evolution. The major results of shock is to convert atomic C, N, O into various molecules. Abundance evolution of SO, HS, H2S, CS, S, S2 and H2CS are given for Vshock = 5, 10 and 15 km/s.
- (Friberg et al., 1988A&A...195..281F)
Facts: They report the first detection of CH3OH in three dark clouds, TMC-1, L134N and B335.
Results: CH3OH lines in TMC-1 show signatures of non-LTE excitation. CH3OH has Ncol ~ 10^13 cm^-2, although less abundant than that of H2CO, but more abundant than that of CH3CHO.
- (Neufeld & Dalgarno, 1989ApJ...340..869N)
Physical and chemical properties of a fast, D-shock are discussed. Fast dissociative shock (D-shock) is faster than 50km/s. H2 and CO dissociation is considered. After the reform of H2, neutral species OH, H2O, O2, CO, CN, HCN, N2, NO, SO and SiO reach high abundances. The molecular ions HeH+, OH+, SO+, CH+, H2+ and H3+ are produced when the gas is hot. The ion emission provide a possible diagnostic of fast shocks.
(tab: column densities of species in the postshock region warmer than 200K)
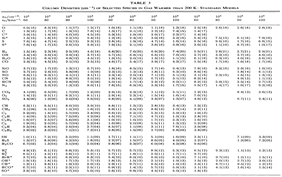
- (from Herbst & Leung, 1989ApJS...69..271H)
Gas-phase chemistry in dense molecular clouds: the pseudo-time-dependent gas-phase chemical model with complex hydrocarbons and organo-nitrogen species included showes that the gas phase (ion-molecule) reactions can produce large amounts of complex molecules at the early stages of the cold clouds only. The early time model abundances, particularly that of CnH, agree with the observations towards cold cloud TMC-1quite well, while the steady state models do not. However, the peak abundances of cyanopolyynes show a slightly discrepant tendency with increasing molecular weight. In the low metal early cloud model with standard (high) rates, several example abundances are [SiO/H2] = 7.4x10^-10;[SO/H2] = 1.7x10^-9; [SO2/H2] = 7.6x10^-10; [CH3OH/H2] = 2.3x10^-9.
(Tabs: Calculated relative abundances from early state models. "high metal" means the set of initial elemental abundances with normal depletion; "low metal" means that with additional 2 orders of magnitude depletion of S, Si, Na, Mg and Fe. The low-metal abundance set usually can reproduce dense cloud chemistry better. "Standard rates" (= high rates) are inverse-squarely temperature dependent ion-polar neutral reaction rates, while low rates are temperature independent ion-polar neutral reaction rates.)
- (Turner et al., 1992ApJ...399..114T)
In order to test shock chemistry, they performed single dish and BIMA observation of multiple transitions of some molecules in the shocked clump IC 443G in the SNR IC 443 and have revealed two groups of molecular species:
......Group 1: CO, HCO+, HCN, CN; they arise in Region 1 with density n1 ~ (2-8)x10^5 cm^-3, T1 < 100K, and size L1 ~ 7x10^17 cm;
......Group 2: SiO, CS, SO, H2CO; they arise in Region 2 with
density n2 > 10^6 cm^-3, T2 = 200-300K, and size L2 < L1.
The group 1 species can be well explained by D-shock model (D = dissociative), while group 2 species can be well interpreted by ND-shock model (ND = non-dissociative). Compared with quiescent cloud abundances, that of group 1 species are not affected by shocks, while that of group 2 species allhave been reduced below the quiescent values. They proposed a physical picture for IC 443G: the shock velocity starts to increase from the rear edge of the clump along the line of sight, increases to a maximum in the D-shock region 1, then decreases to a value consistent with ND-shock in region 2 near the face edge of the clump. The required density gradient should preced the shock.
- PDR chemistry
- (Caselli et al., 1993ApJ...408..548C)
They studied the effects of different thermo-dynamical history of different parts of the same star forming cloud Orion -KL on the chemistry thereof. Two components are considered: a spherical hot core around IRc2 source and a spherical outer compact ridge around the hot core.
The Hot Core condition:
warm Tk ~ 200K,
compact size of < 10",
dense n > 10^7 cm^-3,
chemically characterized by unusually high abundances of H-rich complex N-bearing species (CH2CHCN, CH3CH2CN, etc.
);
The Compact Ridge condition:
warmer Tk ~ 100K than the rest of the Orion Ridge,
larger size of 15",
less dense n > 10^6 cm^-3,
chemically characterized by high abundances of O-bearing species (CH3OH, HCOOCH3, CH3OCH3).
Both components consecutively experience a free-fall contraction (20K for the Compact Ridge, 40K for the Hot Core) and a static warming up phase (evaporation of the grain species). The resulting chemical abundance evolution routes are shown in the figures below. The lower temperature of 20K in the collapse phase of the Compact Ridge model allowed effective adsorption of volatile species such as CO, H and H2 on the grain surface to induce saturation processes to form very abundant unreactive species such as H2O, CH4, NH3, CH3OH and H2CO on the grain surface, which in the next stage of grain evaporation results in the release of large amount of these species into the gas phase chemistry. On the contrary, the higher temperature of 40K in the collapse phase of Hot Core model result of the lack of CO, H and H2 on the grain surface, which allowed the direct reaction among heavier elements to form more complex multi-carbon N- and O- bearing species on the grain surface.
(figs: Evolution of chemical abundances in different stages of the two regions.
........(1st row: Compact Ridge in collapse phase) left -- grain surface species; right -- gas phase species;
........(2nd row: Hot Core in collapse phase) left -- grain surface species; right -- gas phase species;
........(3rd row: Compact Ridge and Hot core after grain evaporation) left -- gas species in Compact Ridge; right -- gas species in Hot Core.
)
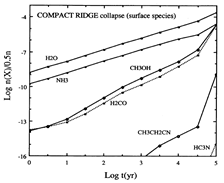 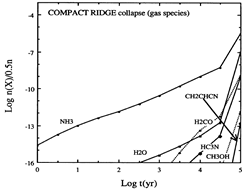
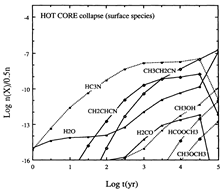 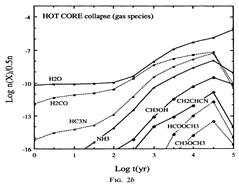
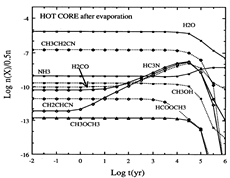
- (Lee et al., 1996A&AS..119..111L)
They give chemical abundances of selected atomic and molecular species in three interstellar cloud models: new standard model, new neutral-neutral model and model 4, for 3 different density: 10^3, 10^4 and 10^5 cm^-3. "Low metal" elemental abundance is used for all the models while "high metal" is also run for the new standard model. The data can be queried in Vizier here.
- (Bachiller & Perez Gutierrez, 1997ApJ...487L..93B)
They present multi-molecular line observations to multiple positions of the low luminosity (11 Lsun) outflow source with processing bipolar jets, L1157. It is shown to be a chemically active outflow source. The blue side of the S-shaped outflow interacts with ambient clouds to induce rich shock chemistry. Thus many lines show extended blue profiles while the red wing is very weak due to the lack of obstruction by interstellar clouds .
(figs.: left -- the observed positions on the CO outflow map; right -- the observed blue lobe line profiles.)
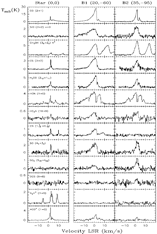
- (Walmsley et al., 1999A&A...342..542W)
They considered Si chemistry in photon-dissociation regions (PDRs) to interpret the observational paradox in Orion Bar: the fine structure emission of Si+ is strong while the SiO emission is missing. The conclusion is that Si in the deep core of the Orion Bar cloud (with Av > 3-4 mag from the surface) is locked in the dust, while in the surface layers the Si is photo-desorbed into gas phase mainly in the form of Si+. It was also found that grain polar ice mantles are probably destroyed by photo-desorption, while thermal evaporation destroys the apolar components of the ice mantles deep in the clouds.
|
Catalogues of SFRs (back to top)
- (also see my source list)
- ---------------------- massive SFRs ---------------------
- (Palla et al., 1991A&A...246..249P)
They selected a sample of 260 cold molecular clouds that could be massive SFRs on the basis of their IRAS colors (limited to |b|<=10deg, DEC>-30deg). 125 of them are classified to be associated with UCH II regions ([25-12]>0.57 and
[60-12]>1.3, Wood and Churchwell, 1989ApJ...340..265W), while the others are considered as at even earlier stages..
- ---------------------- low mass SFRs ---------------------
|
Examples of SFRs
(back to top)
- ---------------------- low mass SFRs ---------------------------
- IRAS 12419-5414 (= Boomerang Nebula ) -- a low mass SFR.
- TMC 1 -- a well studied low mass star formation dark cloud.
- Pipe nebular -- a nearby dark cloud of very early stage of low mass star formation. (Frau et al., 2010ApJ...723.1665F)
- ---------------------- high mass SFRs ---------------------------
- IRAS16547-4247 -- a massive SFR (with an O-type protostar) with possible infalling motion and high mass accretion rate (10^-2 Msun/yr). (see eg., Garay et al., 2007A&A...463..217G)
- IRAS 17441-2822 (= Sgr B2 ) -- a giant molecular
cloud.
- IRAS 19078+0901 (=W49A) -- the most massive and luminous star-forming region in our Galaxy, associated with a H II region.
- IRAS 20126+4104 (=GAL 078.12+03.64 =OH 78.12 +3.63) -- a prototypical source of later early stage of massive SF (high IRAS state) (Fontani et al., 2010A&A...517A..56F)
- IRAS 23385+6053 (=GAL 114.53-00.54) -- a prototypical source of very early stage of massive SF (low IRAS state) (Fontani et al., 2010A&A...517A..56F). (But why is it a H II region in SIMBAD? From here we can see that the classification of evolutionary status is in mess.)
- Gal 24.78+0.08 -- a massive SFR (protostellar mass of 20 Msun) with possible infalling motion and high mass accretion rate (10^-2~10^-4 Msun/yr). (see eg., Beltran et al., 2006Natur.443..427B)
- NGC 7538-IRS9 -- a massive SFR (gas mass of 100-300 Msun) with possible infalling motion and high mass accretion rate (10^-3 Msun/yr). (see eg., Sandell et al., 2005ApJ...621..839S)
- Orion KL -- a giant molecular complex
- W51e2 -- a massive SFR (gas mass of 200 Msun) with possible infalling motion and high mass accretion rate (10^-3 Msun/yr). (see eg., Zhang & Ho, 1997ApJ...488..241Z; Ho & Young, 1996ApJ...472..742H)
- W51 North -- a massive SFR (protostellar mass of 40 Msun) with possible infalling motion and high mass accretion rate (4~7x10^-2 Msun/yr). (see eg., Zapata et al., 2008A&A...479L..25Z)
- ---------------------- other SFRs ---------------------------
- G14.33-0.64 --
An EGO (extended green objects) showing two components in its SiO 6-5 line profile.
- IRS
8* -- The youngest known main sequence star,
discovered in August 2006. It is estimated to be 3.5 million years old [3]. (see its wikipedia
entry)
- L1014 -- An
incredibly faint embedded object representative of a new class of sources
that are only now being detected with the newest telescopes. Their status is
still undetermined, they could be the youngest low-mass Class 0 protostars
yet seen or even very low-mass evolved objects (like a brown
dwarf or even an interstellar planet).
(see its wikipedia entry)
- MWC
349 (= V1478 Cyg) -- it was first discovered in 1978, and is estimated to be only 1,000
years old. (see its wikipedia
entry)
-
VLA1623 -- The first exemplar Class 0 protostar, a type of embedded
protostar that has yet to accrete the majority of its mass. Found in 1993,
is possibly younger than 10,000 years. (see its wikipedia
entry)
|
|